Neuronal Mechanisms for Information Transmission
How neuronal signals encode information
Key Takeaways
The overall function of the nervous system is to organize and control behavior and regulate many internal functions.
Divisions of the nervous system
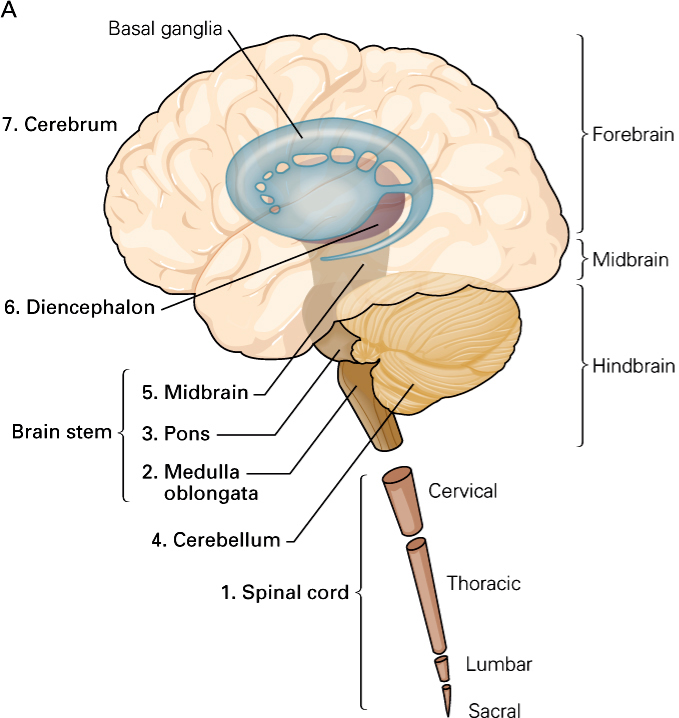
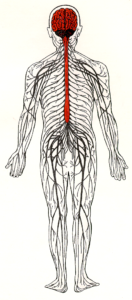
The nervous system has two major divisions: the central nervous system (CNS) and the peripheral nervous system (PNS). The CNS consists of the brain and spinal cord. Its function is to coordinate and transmit signals to the muscles and glands in order to produce movements and adapt the body to changes in the environment. The PNS relays information from peripheral receptors to the CNS and executes motor commands that are generated in the CNS. The PNS includes the nerves of the autonomic division, which innervate the internal organs, such as the heart, the smooth muscles of the body (e.g. blood vessels, intestines), and the exocrine glands (e.g. pancreas, adrenal medulla) and the spinal and cranial nerves of the somatic division, which innervate the skin, the joints, and the skeletal muscles.
Neurons
Key Takeaways
Neurons are considered the building blocks of the CNS, that is, they are its smallest functional units.
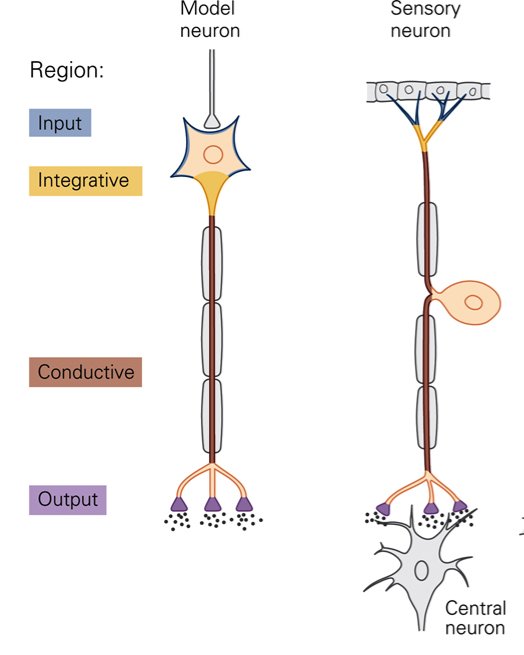
The nervous system is composed of cells, as is the rest of the body. Neural tissue contains two types of cells: nerve cells (neurons) and glial cells. Neurons have specializations that allow them to produce signals that communicate information. Glial cells are not directly involved in neuronal signaling but provide nutrition and support for neurons. Neurons are the basic signaling units of the CNS. The structure of a neuron can be thought of as having input (dendrites, cell body) and output components (axon, presynaptic terminals). Dendrites are outgrowths of the cell body that, like the cell body, receive signals from other neurons. Each neuron typically has multiple dendrites. The cell body maintains cell life; it contains the nucleus (genetic information) and ribosomes (protein synthesis). The cell body’s role in information transmission is to integrate and process signals. At the site of the axon hillock (initial segment), the cell body gives rise to the axon (nerve fiber). Typically, there is only a single axon per neuron. The axon hillock is the site at which electrical signals (action potentials) are generated because the concentration of voltage-sensitive Na+ channels is greatest at the axon hillock. Conduction of action potentials along the axon provides the mechanism by which information is transmitted with high fidelity over long distances, from the axon hillock to the axon terminals. Axons can be very long. For example, cell bodies of motor neurons that innervate the muscles of the toes are located in the spinal cord whereas the axon terminals are located at the site of the muscles. Neurons use a combination of electrical and chemical signals to encode and transmit information. Neurons come in all different shapes and sizes. Many of the differences in form are related to differences in function. Sensory receptors are morphologically specialized to transduce mechanical, thermal or chemical energy into neural impulses (action potentials).
Transduction
Transduction is the process whereby a sensory neuron transforms a physical stimulus (for example, stretch of a muscle) into electrical activity in the cell. The structure of a sensory neuron can be thought of as having distinct input and output components, each of which produces a characteristic signal. When stimulated, the membrane potential in the input component of the sensory neuron changes to generate a so-called receptor potential. Receptor potentials are graded potentials, i.e. the amplitude and duration of the potential are proportional to the amplitude and duration of the stimulus. Receptor potentials are local changes in membrane potentials that are conducted passively. Passive conduction is not suitable for transmission of information over long distances. In order to ensure high fidelity transmission over long distances a second stage of encoding must occur. The change in membrane potential at the input is transformed in action potentials that are propagated over long distances.
Resting membrane potential
The electrical forces that result from the separation of ions across the neuron’s membrane create an electrical potential called the membrane potential. The membrane potential of a neuron that is not conducting neural signals is called the resting membrane potential.
Watch: Resting membrane potential – Narrated Animation & Quiz (3:56)
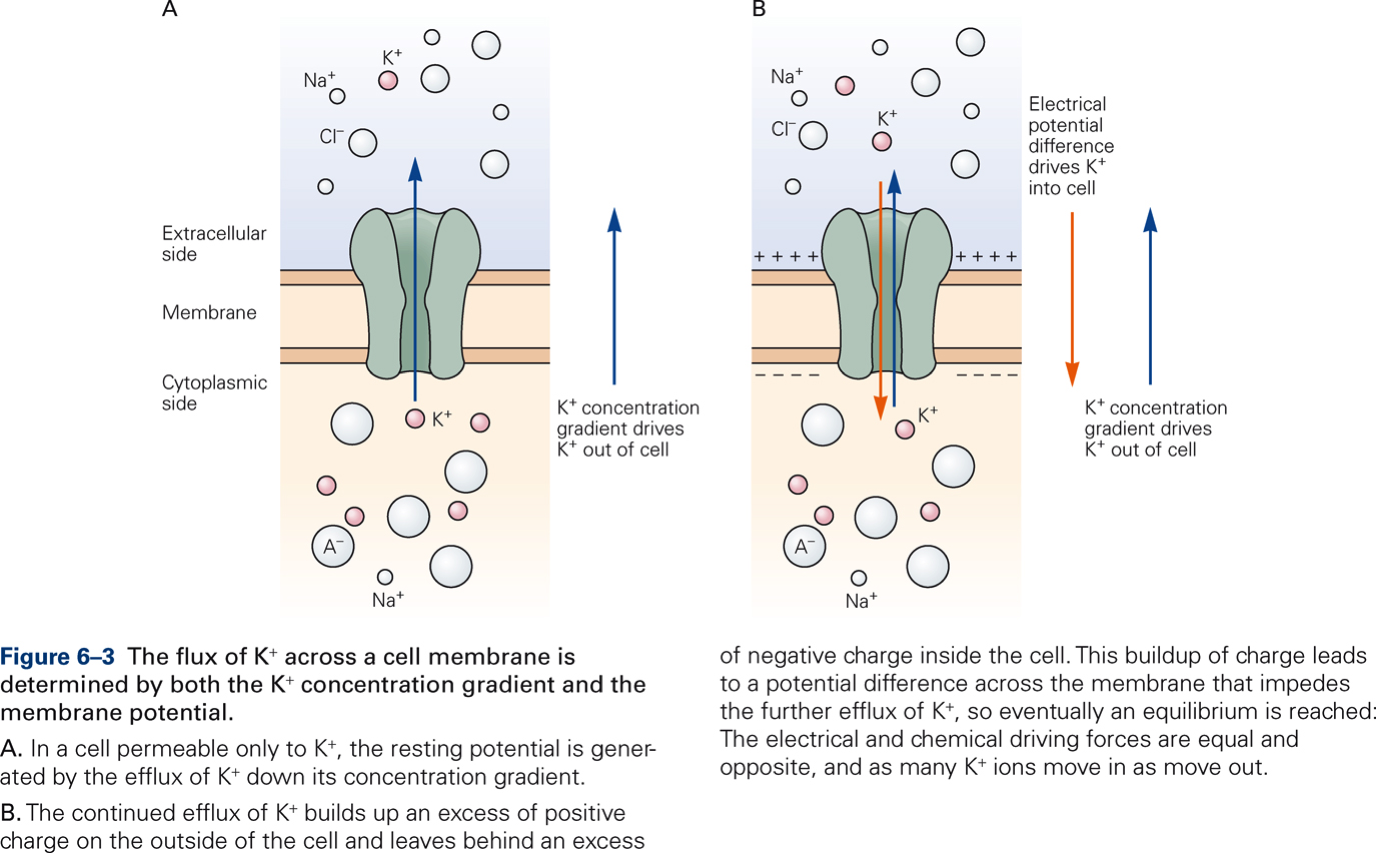
[Bear, M. F., Connors, B. W., & Paradiso, M. A. (2015). Neuroscience: Exploring the Brain (4th ed.). Philadelphia: Wolters Kluwer].
Ions move across the membrane through ion channels. There are two types of ion channels: resting and gated channels.
Resting channels are always open and are not influenced by external factors. They are primarily important for maintaining the resting membrane potential. The resting potential is determined by two factors:
- Concentration gradients of ions across the membrane
- Membrane permeability, which is determined by a) the ease with which the ions can pass through their channel and b) the number of channels
Gated channels are closed when the membrane is at rest. They open and close in response to external signals such as stretch of the membrane, binding of neurotransmitters (ligands), or changes in membrane potential.
All electrical signals are brief changes in resting membrane potential due to the flow of electrical current that results from movement of ions across the membrane. There are two types of electrical signals: graded potentials and action potentials.
Graded Potentials
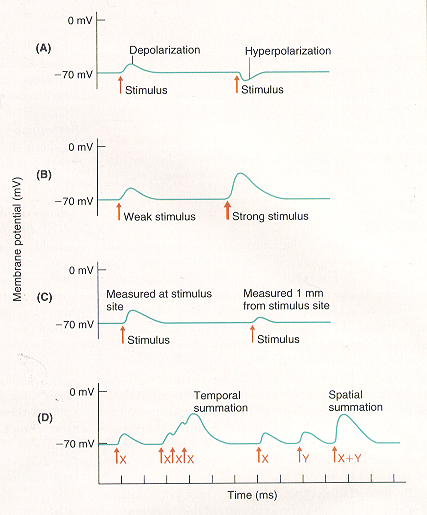
Graded potentials represent a neuron’s input signals. Graded potentials in stretch-sensitive sensory neurons are also called receptor potentials. Graded potentials that result from opening or closing of ion channels by binding of neurotransmitters are called synaptic potentials.
Graded potentials share several characteristics:
- Depolarizations make the membrane potential more positive; hyperpolarizations make the membrane potential more negative.
- Amplitude is graded with intensity of input signals: stronger input stimuli produce larger potentials than weaker stimuli (amplitude varies from 0.1-10 mV).
- Graded potentials are pure local signals that are restricted to the sites where the ion channels that generate them are located. Spread of graded potentials across the neuronal membrane is passive. Amplitude of graded potentials decreases with distance.
- Individual graded potentials can sum to produce potentials of larger amplitude. Spatial summation occurs when two different graded potentials simultaneously activate different sites on a neuron; temporal summation occurs when the same site is activated at slightly different times.
Action potentials
In contrast to graded potentials, action potentials are part of a neuron’s output signals. Action potentials are generated by voltage-sensitive channels, which open and close in response to changes in membrane potential. These channels are concentrated in the region of the axon hillock, and therefore action potentials are initiated there. Action potentials are electrical signals that are brief (~1 ms) and of large amplitude (~100 mV).
Watch: Action Potential – Narrated Animation
[Bear, M. F., Connors, B. W., & Paradiso, M. A. (2015). Neuroscience: Exploring the Brain (4th ed.). Philadelphia: Wolters Kluwer].
Ionic basis of the action potential
When the membrane potential is depolarized and reaches a level called threshold (usually ~ 15mV more positive than resting level), voltage-sensitive Na+ channels open up, Na+ ions rush into the cell and cause a further depolarization of the membrane. This in turn increases Na+ permeability, which results in more depolarization. The positive-feedback relation between membrane depolarization and increased Na+ permeability leads to the rapid depolarization phase of the action potential, which produces a large amplitude action potential in a very short time.
At the peak of the depolarization wave, voltage-sensitive Na+ channels are inactivated and voltage-sensitive K+ channels open up. Rapid outflow of K+ ions returns membrane potential to resting levels (repolarization).
The Na+/K+ pump actively restores ion concentrations to original levels.
Refractory periods
Absolute refractory period: period (~0.5 ms) immediately following the action potential. A second action potential cannot be generated regardless of stimulus intensity due to inactivation of voltage-sensitive Na+ channels.
Relative refractory period: period (4-8 ms) immediately following the absolute refractory period. A second action potential can be generated but only by larger than normal strength stimuli. During the relative refractory period the membrane is hyperpolarized due to activation of voltage-sensitive K+ channels.
Frequency coding
Once threshold is reached, membrane events are no longer dependent on stimulus strength, so that threshold stimuli and suprathreshold stimuli produce the same amplitude action potential. Stronger stimuli are coded in terms of increases in the frequency at which action potentials are generated. This is primarily because a stronger stimulus can excite an axon in its relative refractory period whereas a weaker stimulus cannot. The conversion of stimulus intensity in frequency of action potentials is called frequency coding. Frequency coding is the major way information is communicated in the CNS.
All-or-none law
If a stimulus is strong enough to depolarize the membrane to threshold at the axon hillock, an action potential starts and travels the entire length of the axon (all-or-none law) at a self-sustaining constant amplitude (analogous to pushing the lever to flush the toilet).
[Bear, M. F., Connors, B. W., & Paradiso, M. A. (2015). Neuroscience: Exploring the Brain (4th ed.). Philadelphia: Wolters Kluwer].
Impulse conduction along the axon
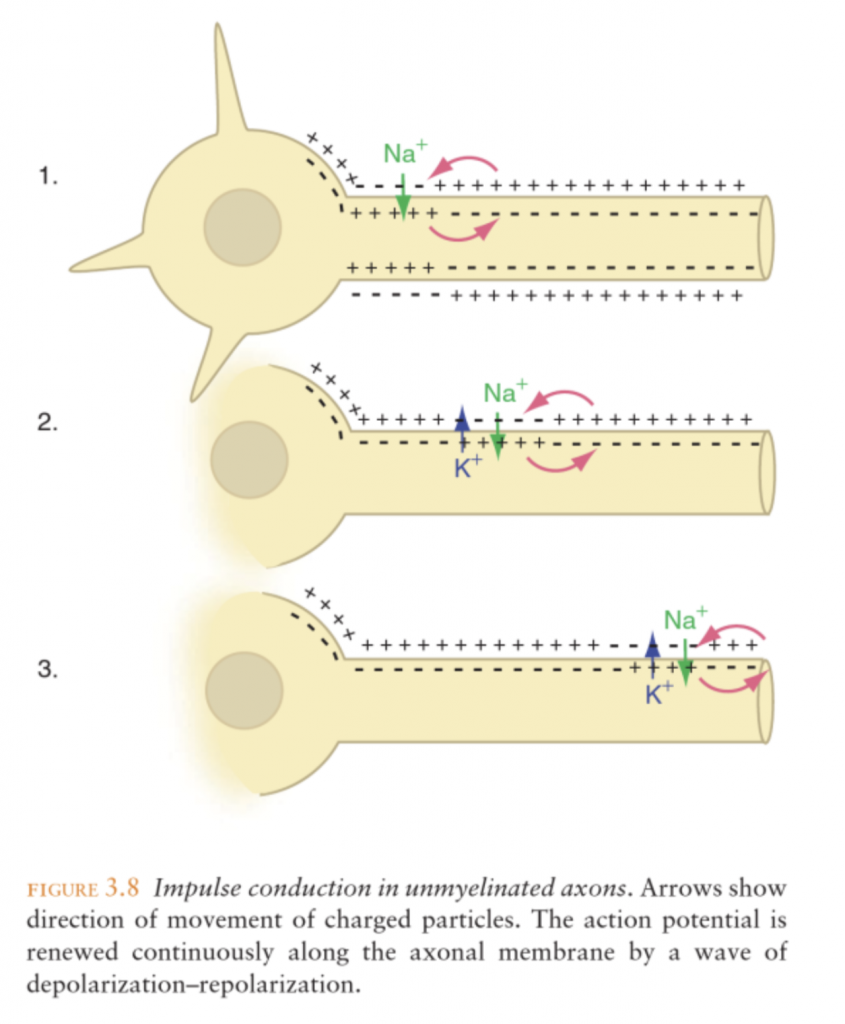
The action potential is regenerated (amplified) as it moves along the axon. Threshold depolarization triggers an action potential at the axon hillock. The action potential travels along the axon and causes a depolarization of a nearby site. When threshold is reached at this site, a new action potential is generated there, etc.
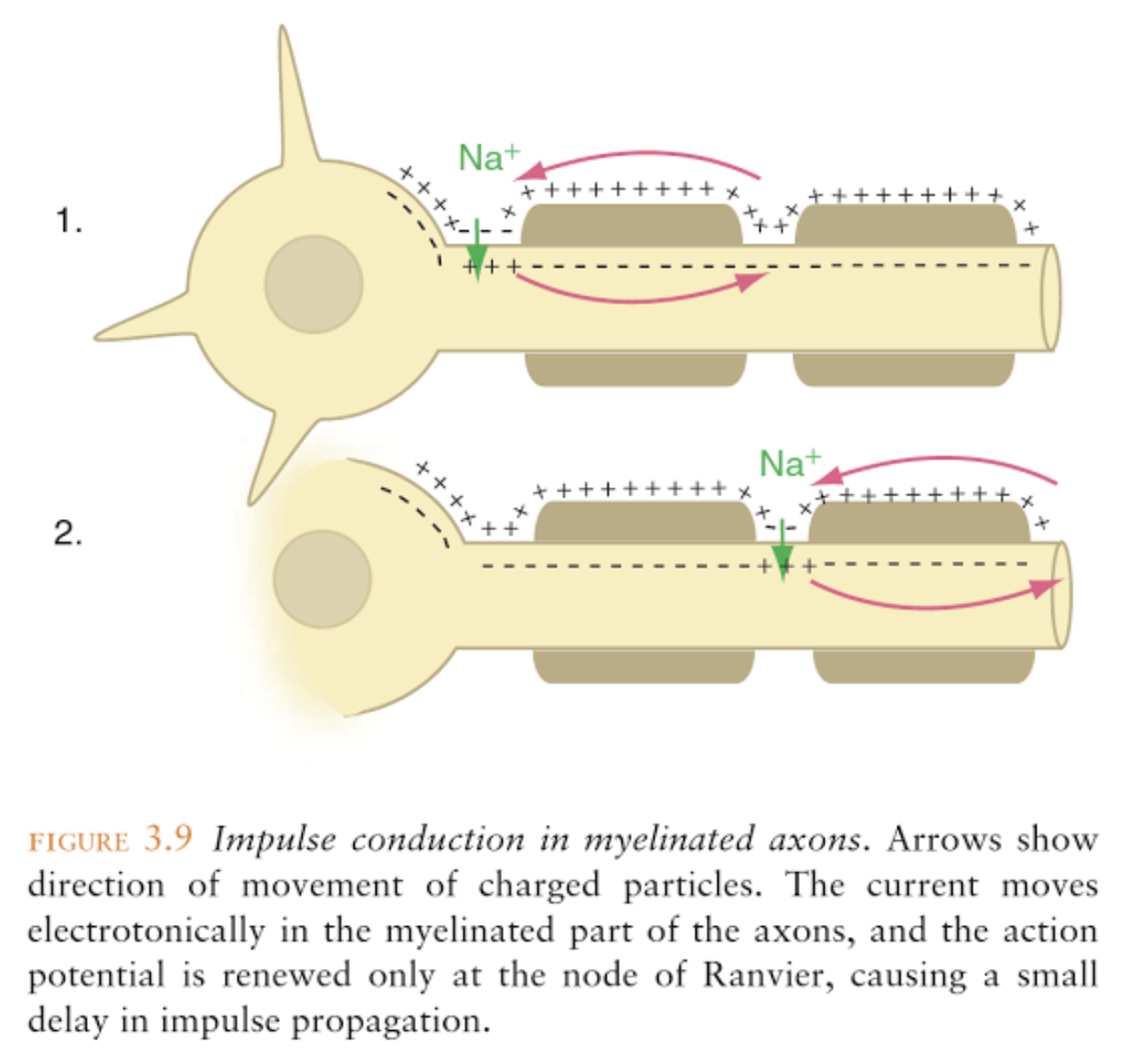
Conduction velocity is increased by either a larger axon diameter or myelination, or both. The myelin sheath is formed by Schwann cells (PNS) or oligodendrocytes (CNS). The myelin sheath is interrupted every mm or so by gaps called nodes of Ranvier. At the nodes, regular membrane depolarization can occur. Saltatory conduction occurs when the depolarization of an action potential at one node spreads passively along the internode regions into the next node where it triggers a full-sized action potential. Saltatory conduction increases the velocity of conduction along the axon and conserves energy by limiting use of the Na+/K+ pump.