M2 | Spinal Mechanisms for Sensorimotor Integration
Somatosensory receptors
Cutaneous receptors
Watch: Receptors in the Skin – Narrated animation & Quiz
The sense of touch is tied to the skin, the most accessible and largest organ in our body. Skin contains a multitude of highly diverse receptors that underlie our sense of touch, pressure, vibration, pain, and temperature. Neural circuits involved in processing information relating to discriminative touch needed for recognizing and manipulating objects, are distinct from circuits that process information pertaining to pain and temperature, which are distinct from circuits that process information about social (affective, pleasant) touch. Large diameter myelinated fibers signal touch-pressure and body position, and transmit this information via the dorsal column-medial lemniscal system to the primary somatosensory cortex. Smaller diameter fibers signal pain and temperature and project via the spinothalamic system to the primary somatosensory cortex. Unmyelinated C tactile fibers signal pleasantness of touch, and they project via the spinothalamic system to the secondary somatosensory (posterior insular) cortex.
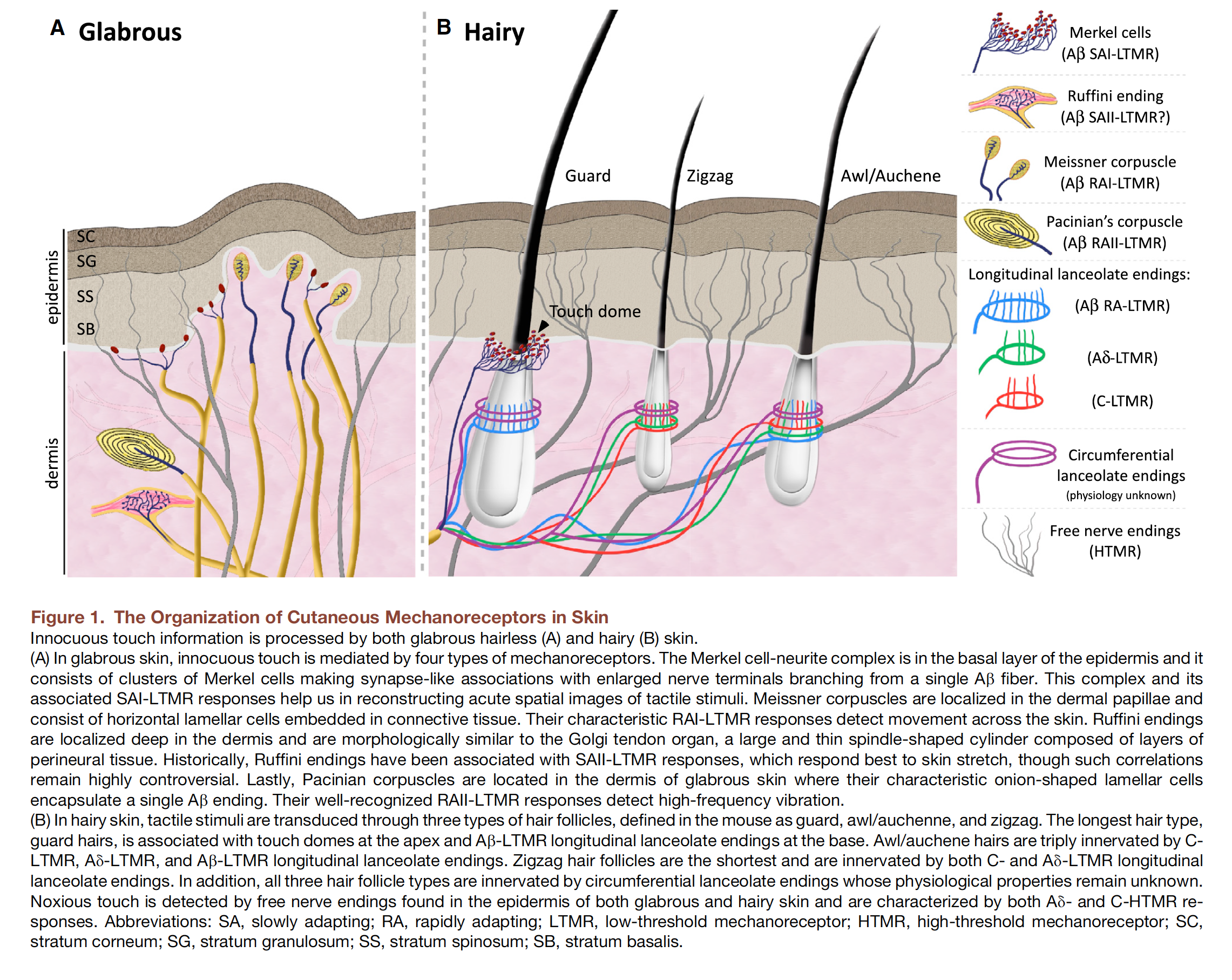
Mechanoreceptors are by definition receptors located in the skin, the muscles, tendons, joints, and deep tissues that transduce mechanical energy associated with specific aspects of touch-pressure applied to the skin into neural signals. Mechanoreceptors vary both in receptive field size and in their rate of adaptation. Five basic types of mechanoreceptors are found in the skin and in subcutaneous layers of fatty and connective tissue. Four types of mechanoreceptors (Merkel disc, Meissner’s corpuscle, Ruffini ending, and Pacinian corpuscle) are found in the glabrous (hairless) skin of the hands, soles of the feet, and the lips. One type (hair follicle receptor) is found exclusively in hairy skin.
Merkel disc
Merkel discs are located deep in the epidermis at the peaks of the primary epidermal ridges. Merkel discs are present at very high densities in the skin of the fingertips and lips and at very low density in hairy skin. They are exquisitely sensitive to small forces that produce skin indentations as little as 50 m. Electrical recordings of single fibers that innervate Merkel discs indicate that they are slowly adapting receptors, i.e. they continue to fire action potentials for as long as the indentation continues.
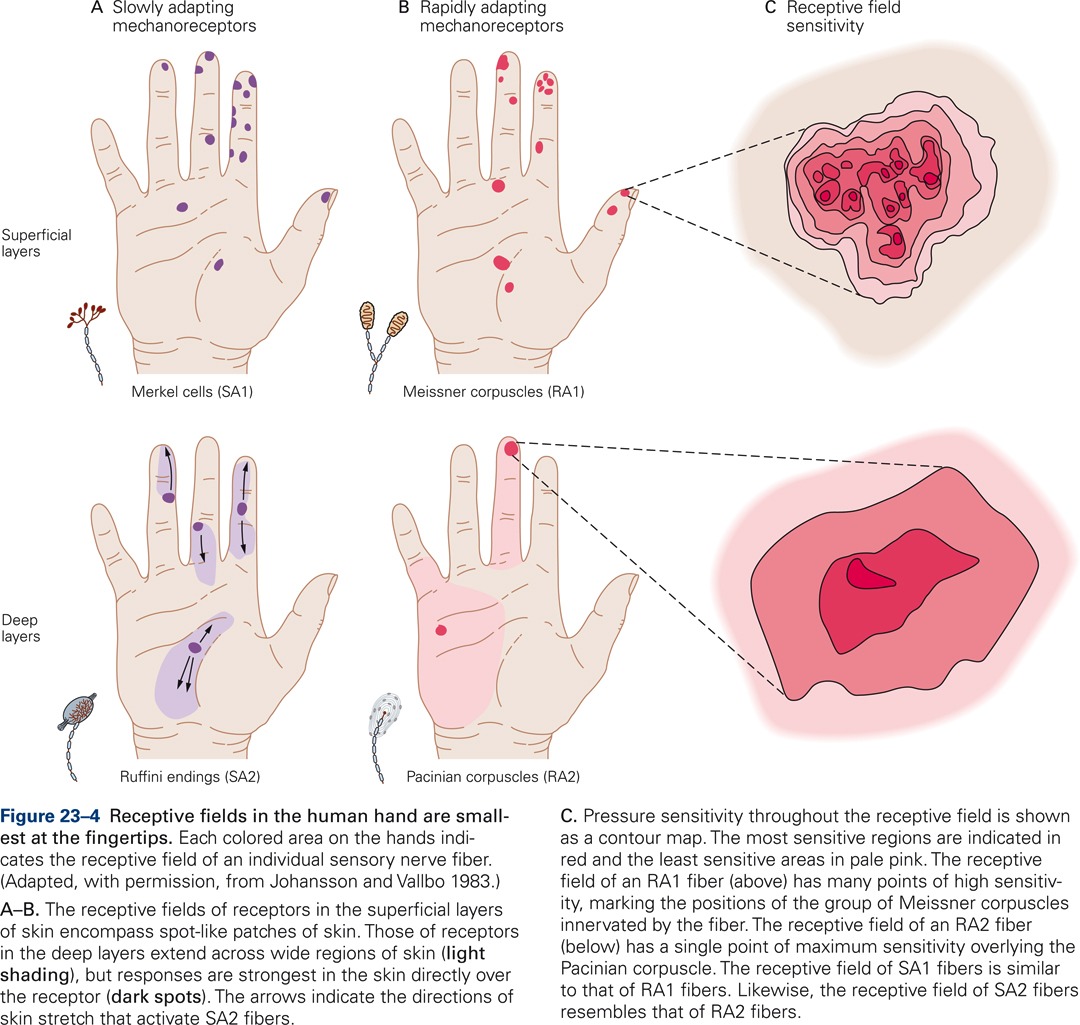
Meissner corpuscle
Meissner’s corpuscles are located in the upper dermis, close to the border with the epidermis. They are present at extremely high densities in the skin of the fingertips; they are not found in hairy skin. Meissner corpuscles are exquisitely sensitive to skin indentation but their ability to localize these movements is modest because a single sensory afferent fiber branches and innervates a group of Meissner corpuscles. This pattern of innervation results in a receptive field characterized by multiple points of high sensitivity, each marking the position of a Meissner corpuscle innervated by the fiber (Fig. 23-4C). Meissner corpuscles are fast adapting receptors, i.e. they respond only at the onset and offset of prolonged skin indentation.
When we grip and lift objects, tiny movements (micro-slips) occur between our skin and the object. Meissner’s corpuscles detect these micro-slips. Signals transmitted to spinal circuits by the primary sensory afferents that innervate Meissner’s corpuscles serve to precisely and reflexively, i.e. without having to think about it, adjust the force of contraction of the relevant finger muscles in order to eliminate the micro-slips. The overall result is that when we grip and lift objects, we do so with the minimal grip force required. The importance of being able to automatically fine-tune grip strength becomes readily apparent when picking ripe raspberries or when lifting an egg.
Ruffini ending
Ruffini endings are located deep in the dermis at relatively low densities. Their longitudinal axis is orientated parallel to the skin surface, which may explain why they are highly sensitive to horizontal skin stretch but relatively insensitive to skin indentation. Ruffini endings are slowly adapting and respond to the amplitude of skin stretch rather than the rate at which it is stretching. Responses are selective for the direction of skin stretch – they may be sensitive for stretch in one direction and be unresponsive for a stretch in the opposite direction as is illustrated by the direction of arrows for the receptive fields on the middle and ring finger in Fig. 23-4. Ruffini endings may serve to provide information about hand and finger position, which is important for controlling digit movements.
Pacinian corpuscle
Pacinian corpuscles are located deep within the dermal layer of skin. Their density is relatively modest: there are about 350 Pacinian corpuscles per finger. Like Meissner’s corpuscles, they are fast adapting receptors, i.e. they only respond at the onset and offset of skin indentation. The receptive field of a Pacinian corpuscle has a single point of maximal sensitivity that overlies the Pacinian corpuscle (Fig. 23-4C). Pacinian corpuscles are exquisitely sensitive to vibration but are poor at localization. They are most sensitive to relatively high-frequency vibration (200-300 Hz). Within this range of frequencies, they can detect skin indentations as small as 10 nm. In addition to detecting skin indentation, Pacinian corpuscles play a role at detecting transient and vibratory stimuli transmitted to the hand by handheld objects like tools or probes.
Hair follicle (hairy skin)
Primary sensory afferent neurons that innervate the hair follicle receptor spiral around the base of the hair follicle or form a lattice-like pattern. Most hair follicle afferents are fast-adapting; they respond to bending of the hair and signal the direction and velocity of objects brushing against hairy skin; they do not respond to sustained deflection of the hair.
Converging evidence from electrophysiological recording, psychophysical studies on people with sensory neuronopathies, and functional brain imaging indicates that affective aspects of touch are signaled by low threshold mechanoreceptive C tactile (CT) afferents (Liljencrantz & Olausson, 2014; McGlone, Wessberg, & Olausson, 2014). C tactile fibers exclusively innervate hairy skin. Their endings wrap around hair follicles, which enable them to respond to deflection of the hairs. CT fibers innervate the small, so-called vellus hairs. Interestingly, vellus hairs are also innervated by Aβ and Aδ fibers. Thus, deflection of vellus hairs gives rise to multiple responses: a fast, emotionally neutral signal mediated by Aβ fibers, and a slow, diffuse signal that conveys pleasant touch mediated by C tactile fibers. Conduction velocities of CT fibers are slow: between 0.6 – 1.3 m/s. Single unit recording has shown that a slowly moving caress strongly activates CT afferents (Loken, Wessberg, Morrison, McGlone, & Olausson, 2009). The greatest firing rates are recorded with caresses that move at velocities in the range of 1-10 m/s (Fig. 3A), which is the same range of stimulus speeds that is associated with maximal sunjective pleasantness ratings in psychophysical studies (Fig. 3B). In fact, firing frequency of CT afferents predicts perceived pleasantness in response to soft brush stroking (Fig. 3C) (Liljencrantz & Olausson, 2014; Loken et al., 2009). Thus, C tactile afferents appear to be tuned to detect a specific type of touch: a light caress with a speed that is optimal for inducing a pleasant sensation.
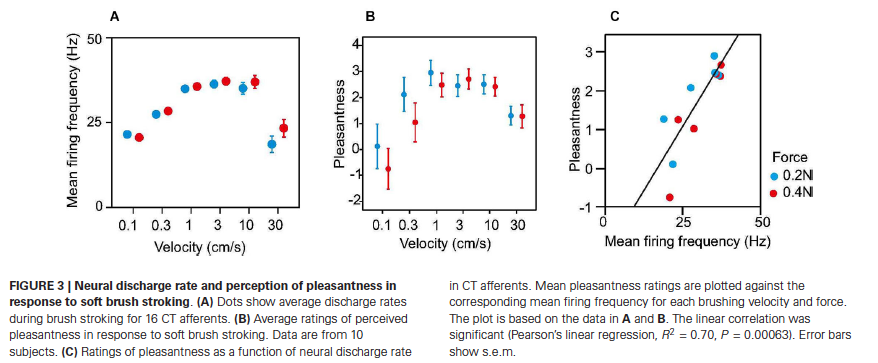
Direct evidence for a role of C tactile afferents in pleasant touch has been difficult to come by because due to the fact that individual hair follicles are innervated by both Aβ and C tactile afferents, CT afferents cannot be activated without also activating Aβ fibers. Patients suffering from peripheral sensory neuronopathy, a rare disorder that selectively destroys the cell bodies of large primary sensory afferents (Sacks, 1985; Sterman Ab Fau – Schaumburg, Schaumburg Hh Fau – Asbury, & Asbury), lack large myelinated Aβ fibers but have intact C tactile fibers. Reexamination of the tactile sensibility of two well studied subjects with peripheral sensory neuronopathy (GL and IW) revealed that both subjects lacked fast, discriminative touch but retained the ability to detect diffuse pleasant touch (Olausson et al., 2008; Olausson et al., 2002). Importantly, the sense of pleasant touch was present in hairy skin but was never found in glabrous skin, indicating that the diffuse sensations were mediated by surviving C tactile afferents, which innervate hairy but not glabrous skin.
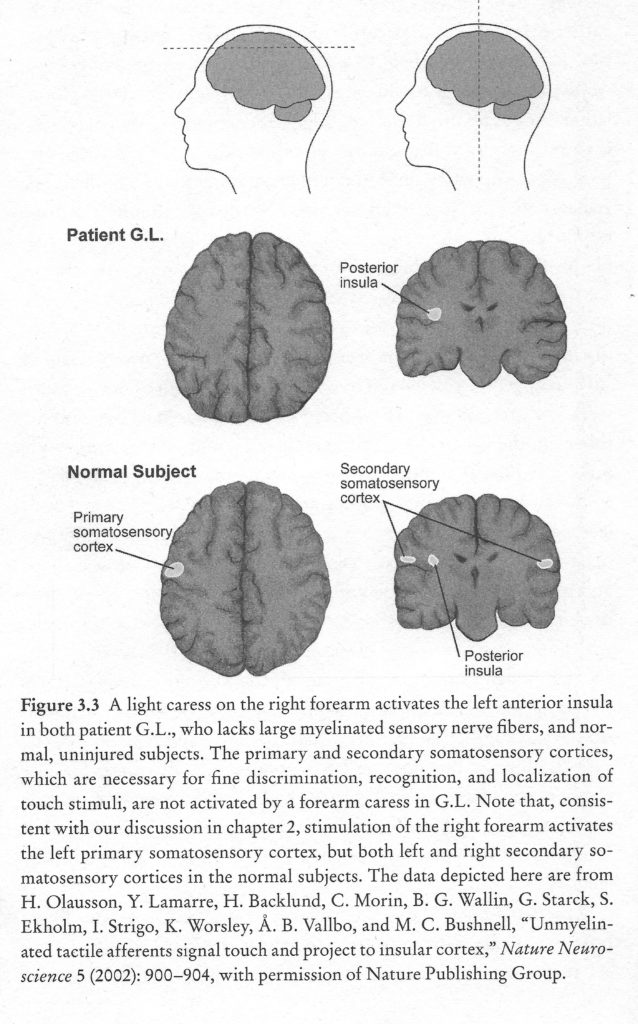
Brain imaging studies in healthy subjects have reported that light brush strokes on the forearm activate primary and secondary somatosensory cortices, which have been implicated in discriminative touch, in addition to the posterior insula, which is involved in emotional aspects of touch perception. Imaging brains of subjects with sensory neuronopathy revealed activation of the posterior insular cortex but not the primary and secondary somatosensory cortices, suggesting that the remaining C tactile afferents activate the posterior insula but not the primary and secondary somatosensory cortices (Olausson et al., 2002).
A genetic mutation in a select group of individuals that reside in Norrbotton, a region of Sweden, north of the artic circle, affects the production of a protein, nerve growth factor beta (NGFbeta), which is critical to the survival of small sensory neurons. People afflicted with the Norrbotton mutation (HSAN-V, hereditary sensory and autonomic neuropathy type V) present with a loss of C tactile fibers and Aδ fibers, and sparing of the larger Aα and Aβ fibers. Thus, carriers of the Norrbotton mutation have essentially the opposite fiber complement than patients with sensory neuronopathy. The Norrbotton population perceives gentle caresses as significantly less pleasant as control subjects, and brain scans indicate only a weak activation of the posterior insula (Morrison et al., 2011).
The combined results are consistent with the view that C tactile afferents innervate hairy skin and function as caress detectors that project to the posterior insula, activation of which makes a critical contribution to the perception of affective (pleasant) touch.
Musculoskeletal receptors
The musculoskeletal system contains five basic types of mechanoreceptors that belong to the large fiber system: muscle spindles in muscles, Golgi tendon organs in tendons, and Ruffini-like and Paciniform receptors, and Golgi endings in joints and ligaments.
Golgi tendon organs (GTOs)
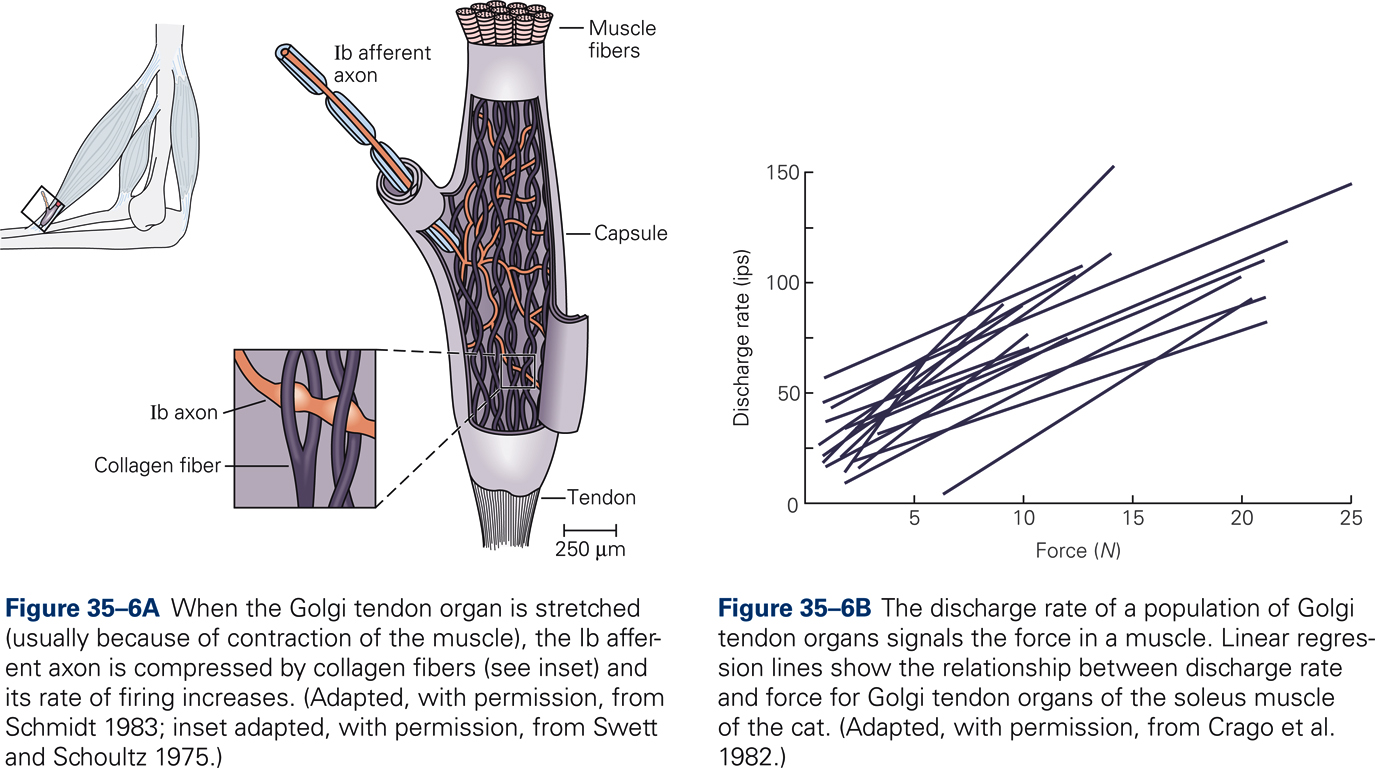
In order to produce accurate movements the CNS needs information about the force of muscle contraction. GTOs provide such information. Information about muscle force is also necessary for maintaining a steady grip on an object and for compensation for muscle fatigue. GTOs are encapsulated structures approximately 1 mm long and 0.1 mm in diameter. They are located between muscle fibers and tendon; they are therefore arranged in series with skeletal muscle fibers. Each GTO is innervated by a single sensory axon, the so-called Ib afferent. Multiple unmyelinated endings of the Ib afferent intertwine with braided collagen fibers. When the GTO is stretched (usually because of contraction rather than lengthening of the muscle), the collagen fibers deform the sensory endings of the Ib afferent neuron, which increases its firing rate. Thus, the GTO is much more sensitive to active muscle contraction than passive muscle stretch. The mean firing rate of the population of GTOs in a given muscle is proportional to the force of muscle contraction, which is consistent with the view that GTOs continuously measure and provide information about the force of muscle contraction.
Muscle spindles
Muscle spindles are sensory receptors in muscles that signal changes in muscle length (stretch) and the rate at which muscle length changes (velocity of stretch). Because muscle length varies with the angle of the joint on which the muscle acts, the brain uses information about muscle length to determine relative positions of body segments.
Muscle spindles are encapsulates structures that are fusiform in shape, i.e. they are shaped in the form of a spindle. Muscle spindles are arranged in parallel with the muscle fibers that are involved in generating skeletal muscle force. They vary in length from approximately 2 to 10 mm, and they are distributed throughout the muscle belly of all skeletal muscles, with the exception of perioral muscles, which move the lips, and the muscles of the inner ear. Their density if particularly high in muscles of the neck and hands.
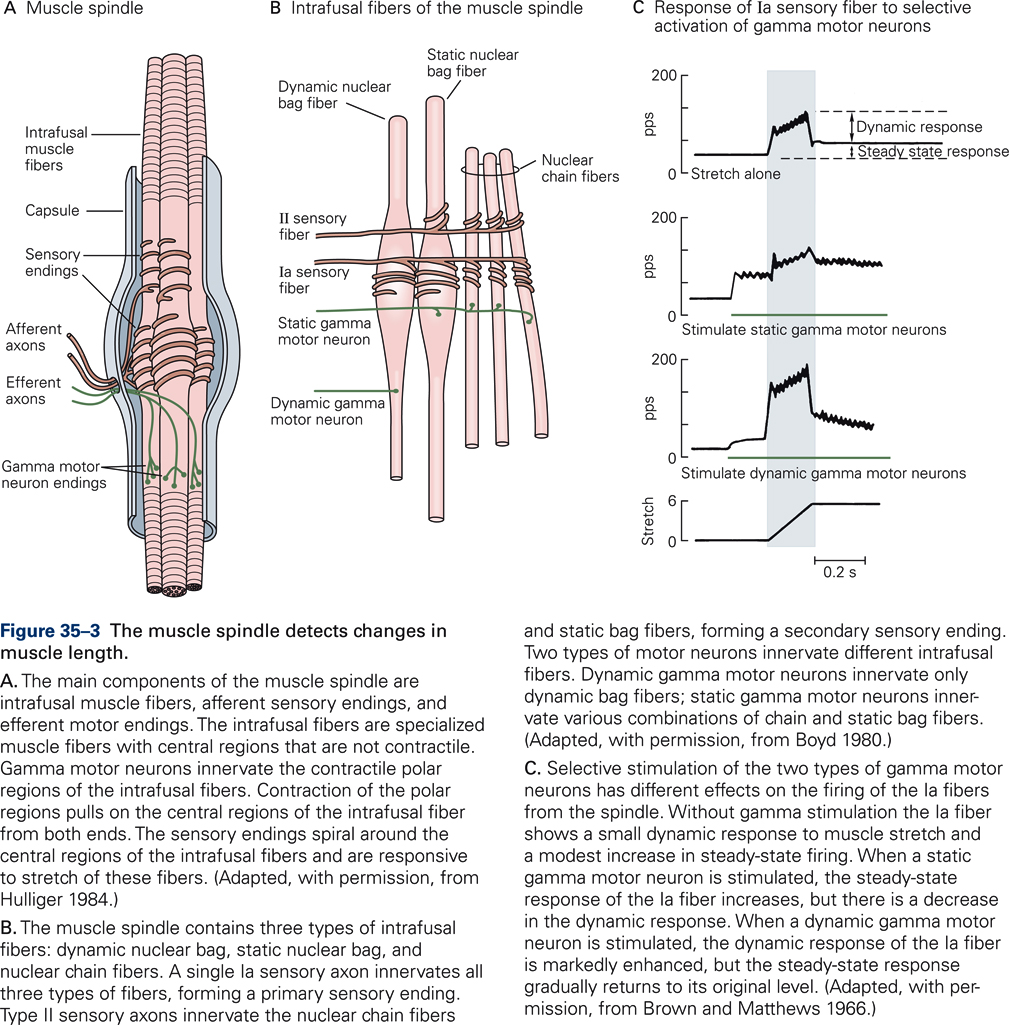
Muscle spindles consist of three components: intrafusal muscle fibers, afferent sensory endings, and efferent motor endings. Intrafusal (i.e. inside the spindle) muscle fibers are specialized muscle fibers with central regions that are non-contractile. Intrafusal muscle fibers do not contribute to the generation of muscle force; they are distinguished from extrafusal muscle fibers, which are the fibers that generate the main force of contraction. Intrafusal fibers differ in their contractile properties. There are two main types of intrafusal fibers: nuclear bag fibers and nuclear chain fibers (Fig. 35-3B).
Each intrafusal fiber is innervated by one or more sensory axons that wrap around the central part of the intrafusal fibers. The sensory afferents increase firing when the central region is stretched, and decrease firing upon release of stretch. Together, all terminations of a single group Ia afferent on intrafusal fibers make up a primary ending, and all terminations of a single group II afferent make up a secondary ending (Fig. 35-3B).
Efferent (motor) axons terminate on the two end regions of the intrafusal fibers. They change the sensitivity of the muscle spindle. These are axons of a special type of motor neurons, the γ-motoneurons, as opposed to the α-motor neurons that innervate the extrafusal muscle fibers and generate the muscle’s contractile force. Increased firing of γ-motoneurons contracts the two end regions of the intrafusal fibers, which stretches the central, length-monitoring region of the intrafusal fiber, thereby increasing the sensitivity of the spindle. We distinguish static and dynamic γ-motor neurons, depending on the specific types of intrafusal fibers innervated by the γ-motor neurons (Fig. 35-3B). Figure 35-3C illustrates the effect of stimulating γ-motor neurons on the discharge pattern of a Ia afferent. Activation of static γ-motor neurons during muscle stretch increases the steady state firing rates of Ia afferents relative to the firing rate without stimulation. During the stretch, the dynamic response is reduced. Stimulation of the dynamic γ-motor neuron results in a small increase in static response but a large increase in dynamic response. Thus, innervation of muscle spindles by independently controlled γ-motor neurons provides the brain with a mechanism to adjust the spindle’s sensitivity and thus fine-tune the information it receives.
When α-motor neurons (i.e. those that innervate extrafusal muscle fibers) contract and the muscle shortens, γ-motor neurons are activated simultaneously to prevent the intrafusal fibers from slackening. The simultaneous activation of α-motor neurons and γ-motor neurons is called α- γ coactivation. α- γ coactivation keeps the intrafusal fiber under tension and maintains the firing rate of the Ia fibers in a range that is optimal for signaling changes in muscle length, regardless of the actual length of the muscle. Thus, α-γ coactivation stabilizes the sensitivity of the muscle spindles — it is characteristic of many voluntary movements.
Joint receptors
Joint receptors are mechanoreceptors that are found in the ligaments and soft tissues of joints. They are of different types: Ruffini-like, Paciniform, and Golgi receptors. Joint receptors are innervated by afferent fibers of similar types as those that innervate cutaneous and muscle receptors, and they have similar adaptation characteristics. Deformation of the joint capsule activates Paciniform and Ruffini-like receptors; stretch of ligaments activates Golgi endings. Recording of joint receptor afferent fibers has demonstrated that the receptors are activated primarily at extreme joint positions, which is consistent with the view that joint receptors are limit detectors that protect joints from hyperextension.
Nociceptors
Nociceptors are sensory receptors that selectively respond to stimuli that damage tissue, such as intense mechanical stimulation, extreme temperatures (heat or cold), oxygen deprivation, or contact with certain chemicals. Nociceptors serve a protective function: they signal impending injury and provide constant awareness of tissues that have been injured and, therefore, need to be protected. Nociceptors are different from other sensory receptors in that they respond in a similar way to more than one kind of energy (e.g. mechanical, thermal, or chemical). Different types of nociceptors produce molecules that are responsible for the response to noxious, that is, painful, mechanical, thermal, or chemical stimuli. The same receptors also respond to certain plant-derived chemicals such as capsaicin that can produce pain.
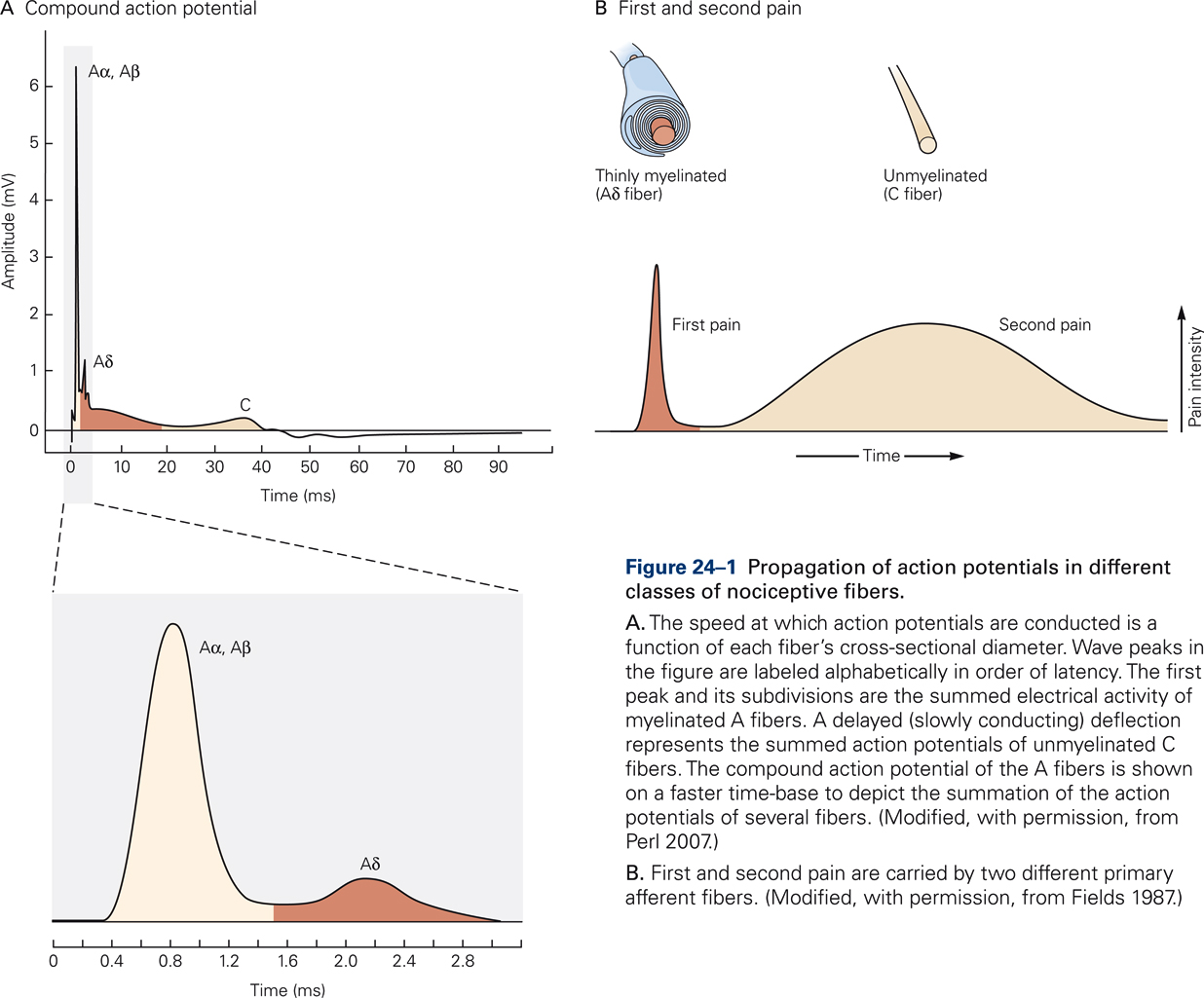
It is important to note that nociception and pain are two distinctly different concepts. Nociception refers to the sensory processes that signal tissue injury or the risk of tissue injury. In contrast, pain refers to the unpleasant experiences and emotions that are associated with tissue damage or the risk thereof. Pain is the feeling or the perception of aching, stinging, throbbing, burning, soreness, etc. that may result from the selective activation of nociceptors. Because pain is a subjective experience, the same sensory stimulus can elicit highly varying responses in the same individual at different times, depending on emotional state and behavioral context. Thus, pain is not the direct expression of a sensory event but rather the product of extensive neural processing of a variety of signals. Understanding the factors that underlie this modulation of pain by internal and external conditions is of great importance for the clinical management of patients with chronic pain.
Nociceptors are present in most body tissues, including skin, bone, muscles, internal organs, and blood vessels, but they are absent in the brain. Most nociceptors in the skin, muscles, joints, and viscera are free nerve endings that are categorized into two main classes depending on the myelination of their afferent fibers (Fig. 24-1). Nociceptors innervated by Aδ fibers transmit short-latency signals of sharp, pricking pain (“first pain”), such as is generated by a sharp object that punctures the skin. The majority of Aδ receptors are referred to as mechanical nociceptors; however, many of these also respond to noxious heat that can burn the skin.
Nociceptors innervated by unmyelinated C fibers provide the neural substrate for dull, burning pain that is diffusely localized and often poorly tolerated (“second pain”). The majority of nociceptors innervated by C fibers are polymodal nociceptors that respond to noxious mechanical, thermal, and chemical stimuli. Pinch or puncture, noxious heat or cold, and irritant chemicals applied to the skin produce long-lasting sensations of intense, burning pain. Visceral nociceptors are primarily activated by swelling and distension and generate intense pain.
Genetic and molecular biological studies of families with congenital insensitivity to pain have revealed mutations in a specific gene, SCN9A, which codes for a particular type of voltage-dependent Na+ channels that are unique to nociceptors. This finding is significant as it not only provides insights in the molecular mechanisms of nociception but it also will result in the development of novel approaches for improved clinical management of pain.
Virtually everyone at some time or other has experienced hypersensitivity to somatosensory stimulation, due to heat or chemical burns, sunburns, abrasions, lacerations or other skin damage. This phenomenon is known as hyperalgesia, and is characterized by enhanced sensitivity of somatosensory receptors induced by tissue damage, and more intense pain sensations. Much has been learned in recent years about structures and mechanisms underlying hyperalgesia. In addition to the direct responses of nociceptors to the stimuli that damage tissue, tissue damage also triggers the release of chemicals at the site of damage, such as certain neurotransmitters (e.g. glutamate, serotonin), peptides (e.g. substance P, bradykinin), lipids (e.g. prostaglandins, endocannabinoids), and other substances, which in combination can cause inflammation. The cardinal signs of inflammation in the skin are pain, heat, redness, and swelling.
Several of these inflammatory agents modulate the excitability of nociceptors, enhancing their sensitivity to thermal or mechanical stimulation. Bradykinin, for example, increases the sensitivity of heat-activated ion channels. Prostaglandins, which are byproducts of the breakdown of lipid membranes, greatly enhance the sensitivity of nociceptors to other stimuli. Prostaglandins also play a role in the clinical condition of allodynia, in which innocuous stimuli result in pain, as when sunburned skin is touched. Persistent injury can result in permanent changes in neural circuitry that amplify and prolong pain and result in a state of hypersensitivity to pain. In these cases, innocuous stimuli may result in pain. Aspirin and other non-steroidal anti-inflammatory drugs are effective in treating hyperalgesia and allodynia because they inhibit the enzymes required for prostaglandin synthesis.
Central and peripheral influences on pain sensitivity
Central influences associated with emotional states or behavioral contexts, such as stress, fear, anxiety, motivation, determination, or mind set are able to modulate pain sensitivity. For example, soldiers or athletes who sustain injuries may not feel pain until the battle or game is over. Central influences that suppress pain arise from several brain regions. One important source of descending projections originates from structures in the midbrain: the periventricular and periaqueductal gray matter (PAG). Serotonergic PAG neurons project to the dorsal horn of the spinal cord, where they inhibit the primary afferent neurons that transmit nociceptive signals. Electrical stimulation of the PAG region is clinically relevant as it produces a powerful analgesia without affecting sensitivity to touch, pressure, or temperature.
Pain sensitivity is also regulated by afferent (ascending) projections of low-threshold, non-nociceptive mechanoreceptors. , Pain is reduced when large-diameter fibers of group I (Aα) and group II (Aβ) varieties, which carry touch and proprioceptive information, are activated in conjunction with the small-fibered nociceptive system (C fibers). Presumably, this explains why shaking or applying pressure on a finger hit by a hammer suppresses pain, or why gentle stroking of the abdomen is effective in reducing pain during childbirth.
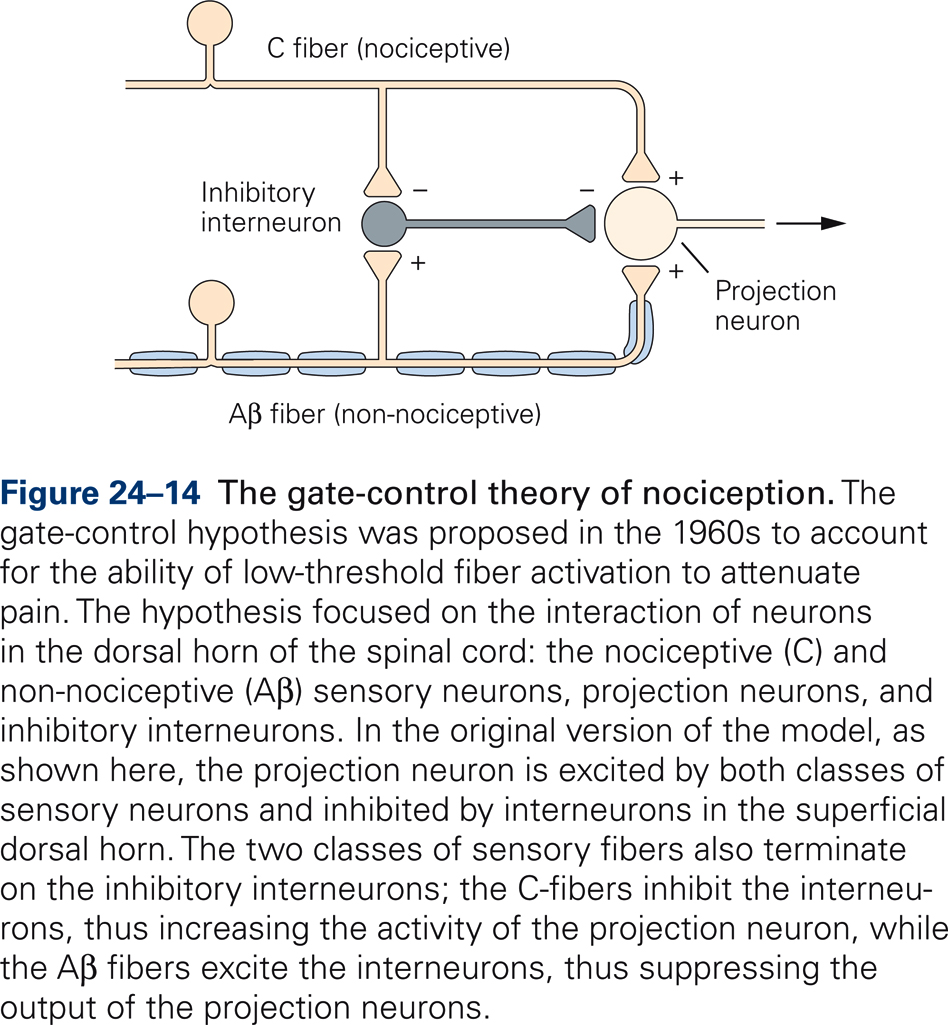
The gate-control theory of pain (Melzack & Wall, 1965) hypothesizes a mechanism that can explain these observations (Fig. 24-14). Projection neurons, which transmit nociceptive information centrally, receive inputs from both large-fibered mechanoreceptors and small-fibered nociceptors. In addition, projection neurons receive input from inhibitory interneurons that are excited by the large-diameter mechanoreceptors and inhibited by the small-diameter pain fibers. Thus, the projection neurons are maximally excited by selective activation of nociceptors. Concurrent activation of large-diameter mechanoreceptors, however, activates the inhibitory interneurons, thereby reducing the central transmission of nociceptive signals.
Itch
Itch is a common somatosensory experience that has a number of characteristics in common with pain (nociception). Itch is an unpleasant experience regardless of intensity, and we attempt to relieve itch by scratching. Itch is usually a temporary condition of minor annoyance; however, itch can also manifest chronically due to allergies, stress, or certain psychiatric conditions. Chronic itch can be as debilitating as chronic pain, and its clinical management is notoriously difficult with treatment and drugs presently available.
Itch can be induced by intradermal injection of histamine or by release of endogenous histamine, which suggests that transduction involves histamine receptors. For example, temporary itch induced by an insect bite or poison ivy is caused by allergens entering the skin. Allergens elicit an immune response by activating mast cells, which, in turn, release histamine. Histamine binds with protein receptors on primary sensory afferent neurons in the skin. Through a cascade of events the primary sensory afferents transmit an itch signal to the spinal cord and brain. Anti-histamines prevent the binding of histamine to the receptors, thereby preventing the transmission of an itch signal. Not all itch is mediated by histamine, however. Itch can also result from various endogenous and exogenous substances that react with itch-inducing receptors or ion channels.
Both pain and itch are mediated by small-diameter dorsal root ganglion neurons with unmyelinated axons (C fibers). There is ample evidence for the existence of subsets of nociceptors that are polymodal, responsive to both itch and pain caused by noxious heat or chemicals such as capsaicin. In fact, until recently, itch was thought to results from low firing rate in nociceptors. The observation that scratching an itch makes the itch worse, may be because scratching an itch also causes a small amount of pain, which for a brief moment masks the itchy feeling. In response to the pain signal, the brain releases serotonin, a neurotransmitter that regulates mood and mediates reward. Serotonin, in turn, activates nociceptors that transmit the itch signals and thus sets in motion a vicious cycle: you itch, you scratch, you itch, etc. (Zhao et al., 2014). Observations that intradermal injection of a large dose of histamine produces a long-lasting intense itch but only mild pain, suggest that itch may not be the result of low-level firing in nociceptors, however. Moreover, recent findings indicate the existence of a subset of nociceptors specifically linked to itch (Han et al., 2013). The identification of a specific subpopulation of itch-mediating nociceptors is important because it not only increases our understanding of the mechanisms by which itch signals are generated and transmitted but also opens new avenues for the development of itch therapies.
Thermoreceptors
Humans can distinguish four types of thermal sensations: cold, cool, warm, and hot. Thermal sensations arise from the ensemble activity of multiple types of afferent fibers: low-threshold and high-threshold cold receptors, warm receptors, and two types of heat nociceptors.
Low-threshold cold receptors are small-diameter Aδ fibers with unmyelinated sensory endings that terminate in the epidermis. They are exquisitely sensitive to rapid decreases in skin temperature; sensitivity is much lower to gradual changes in skin temperature. Warm receptors are unmyelinated small-diameter C fibers that terminate in the dermis. They are slowly adapting receptors whose firing rates are proportional to skin temperature up to the threshold of pain. They saturate at higher temperatures. Heat nociceptors are activated at temperatures > 45C. Their output signals are transmitted by both Aδ and C fibers and result in burning pain.
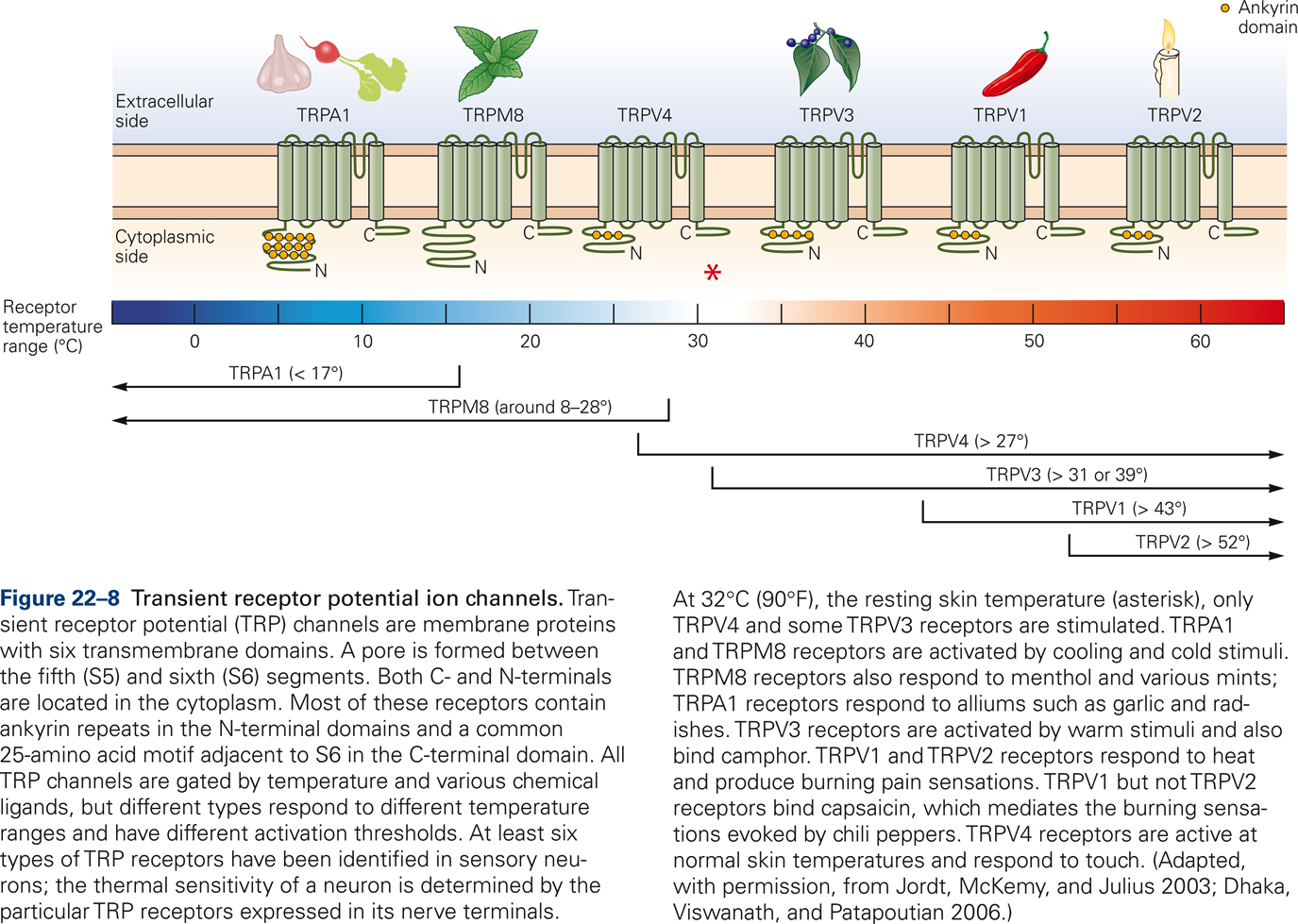
Thermal stimuli activate specific classes of ion channels in the cell membrane; the so-called Transient Receptor Potential (TRP) channels (Jordt et al, 2003). All TRP channels are transmembrane proteins that are gated by temperature and various chemical ligands (Fig. 22-8). Different types of TRP channels respond to different temperature ranges and have different thresholds of activation. For example, TRPA1 and TRPM8 receptors are activated by cooling of the skin. TRPM8 receptors are also activated by menthol and mint. TRPV1 and TRPV2 receptors respond to heat and generate burning pain sensations. TRPV1 receptors also bind capsaicin, which mediates the burning sensations elicited by hot chili peppers.
The discovery and identification of TRP channels, each selectively sensitive to a range of temperatures, varying from cold to burning hot, combined with observations that different TRP channels are gated by specific chemicals, such as capsaicin and mint, are important clinically because they provide opportunities for the development of novel treatments of chronic pain syndromes.