Unit Two
Day 14: Macromolecules
Applying Core Ideas: Making Really Large Molecules
DNA is an example of a macromolecule—a huge molecule. A typical DNA molecule from the human genome is about 4 cm long and contains about 4 billion atoms. Suppose that you want to make a really, really big molecule like this. How might you go about it? What element or elements would likely be involved? What kind of chemical reaction might be used? Construct a model process, based on molecular structure and types of chemical reactions, that would enable synthesis of very large molecules. Write a description of the process in your notebook.
Polymers are large molecules made by covalently linking many, many small molecules. The small molecules that link to form a polymer are called monomers. Polymers can be natural (such as starch and proteins) or synthetic (such as nylon and polypropylene). Because they include a variety of structures and functional groups, polymers have a broad range of properties and uses that make polymer-based plastics integral parts of our everyday lives.
Polymers provide good examples of how we can apply models involving molecular structure, functional groups, and intermolecular attractions to explain and predict chemical and physical properties of materials.
D14.1 Addition Polymers
Addition polymers are made by addition reactions, wherein two molecules combine to form a single product molecule (see examples of addition reactions involving alkenes in Section D7.4). Typical monomers for addition polymerization have at least one double bond.
Figure 1 shows polyethylene, an addition polymer, forming from ethylene (ethene, H2C=CH2) monomers.
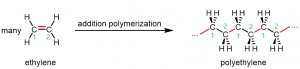
The reaction can be initiated by a molecule with an unpaired electron: a free radical. An example initiator is an organic peroxide, which can form two free radicals when the relatively weak O-O bond breaks:
Activity 1: Analyzing Peroxide Decomposition
When a radical encounters an ethylene monomer, the ethylene π bond breaks. One electron from the π bond goes to pair with the electron from the radical and form a σ bond. The other electron from the π bond remains a radical, and can go on to react with another ethylene monomer.
The process in Figure 2 repeats with many, many more monomers, sometimes as many as 100,000 units, yielding a long polymer chain of carbon atoms.
D14.2 Polymer Structure and Properties
In polyethylene, the addition reactions convert all ethylene double bonds to single bonds and join the monomers without losing any atoms. Hence, this polymer at a molecular level consists of a collection of long-chain alkane molecules, most of which contain tens of thousands of carbon atoms. Many of polyethylene’s properties are what we would expect from this molecular composition.
If the intermolecular forces between the chains are smaller, so that it is easier for the molecules to move past one another, the polymer will be softer and easier to scratch. If the intermolecular forces between the chains are sufficiently strong to prevent motion of the molecules past one another, the polymer will be harder or more rigid. Because polyethylene is a mixture of long alkane molecules, each of slightly different chain length, it softens over a range of temperatures rather than having a single melting point. You may have noticed that some plastics, when wrapped around something in a microwave oven, soften and change shape but never become liquid.
This leads to an important idea in the field of materials science: materials can be “tuned” to give exactly the properties desired. By adjusting the strengths of the intermolecular forces one can obtain plastic materials with a range of properties.
For polyethylene, the extent of branching of a polymer strand can be varied. Depending on the production process, a polymer strand can be a very long linear chain or there can be branching in the chain:
Exercise 1: Polymer Structure and Properties
Polymer properties can also be affected by cross linking. A cross-link is a covalent σ bond between two separate polymer chains that is not at the end of either chain.
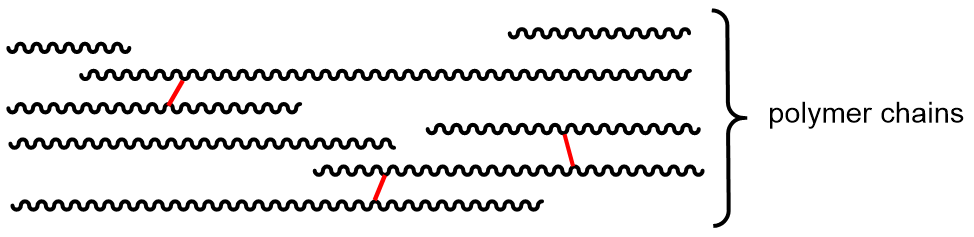
Cross links increase the molecular weight and limit the motions of the chains with respect to one another. With enough cross links, a sample of a polymer can become a three-dimensional network held by σ bonds—a single gigantic molecule. Another way to think about cross links: because they are covalent bonds, they are stronger than intermolecular forces between polymer chains and therefore amplify the effect of increasing intermolecular forces on polymer properties.
D14.3 Various Addition Polymers
Table 1 below lists monomers for some well-known addition polymers and also some of their uses. You can probably find at least one example of each of them in your home. Each monomer is a variation of the ethylene (ethene) structure in which one or more H atoms has been replaced by another group (highlighted in the table). Such substitution(s) in the monomer allow the physical properties of the polymer, such as density, melting range, strength, and hydrophobicity, to be precisely controlled, and the polymer tailored for specialized uses. Note that in the equation below the polymer structure is specified by enclosing a single repeating unit in brackets and specifying the repeating nature by a subscript n.
Monomer | Common Name | Polymer | Some Typical Uses |
---|---|---|---|
![]() |
Ethylene | Polyethylene | Film for packaging and bags, toys, bottles, coatings |
![]() |
Propylene | Polypropylene (Herculon) | Milk cartons, rope, outdoor carpeting |
![]() |
Styrene | Polystyrene (Styrofoam, Styron) | Transparent containers, plastic glasses, refrigerators, styrofoam |
![]() |
Vinyl chloride | Polyvinyl chloride (PVC) | Pipe and tubing, raincoats, curtains, phonograph records, luggage, floor tiles |
![]() |
Vinylidene chloride | Polyvinylidene chloride (Saran) | Clinging food wrap |
![]() |
Acrylonitrile | Polyacrylonitrile (Orlon, Acrilan) | Textiles, rugs |
![]() |
Tetrafluoroethylene | Polytetrafluoroethylene (Teflon) | Nonstick pan coatings, bearings, gaskets |
![]() |
Vinyl acetate | Polyvinyl acetate | Elmer’s glue, wood glue |
![]() |
Methyl methacrylate | Polymethyl methacrylate (Plexiglass, Lucite) | Stiff, clear, plastic sheets, blocks, tubing, and other shapes |
The notation where a repeating unit in a polymer chain is shown in brackets emphasizes that it is important to be able to recognize a repeating unit within a polymer chain. It is also important to be able to generate the full polymer structure from a repeating unit. Enclosed within the bracket can be one repeating unit (equivalent of a monomer), or two, or three, etc. Let’s use polyvinylidene chloride as an example:
Shown on the left is a polyvinylidene chloride polymer strand. The individual repeating units are color-coded. However, there are two ways to consider repeating units: starting at the CH2 (top) or the CCl2 (bottom). Either way is fine.
You can see that the repeating pattern in this polymer is alternating CH2 and CCl2. When you put a bracket around a repeating unit, you are communicating that what is outside of the bracket exactly repeats what is inside, starting immediately with the bonds that are divided by the bracket. Therefore, the leftmost carbon atom of the next repeating unit is directly bonded to the rightmost carbon of the unit shown.
In other words, something like the following is incorrect for depicting polyvinylidene chloride:
It is depicting a polymer where there are CH2 unit bonded to CH2 unit, which does not occur in polyvinylidene chloride. (this polymer is …-CH2-CH2-CCl2-CH2-CH2-CCl2-CH2-CH2-CCl2-…)
Exercise 2: Identifying a Monomer from a Polymer Structure
D14.4 Conjugated Diene Polymers
Conjugated dienes (alkenes with two double bonds and a single bond in between) can be polymerized to form important substances, such as rubber. This occurs in nature as well as in the laboratory. The simplest conjugated diene is 1,3-butadiene; Figure 4 shows the 1,4-polymerization of this monomer. In the resulting polymer, a new σ bond (highlighted in red) is formed between carbon 1 of one monomer and carbon 4 of another monomer, and within each monomer, a π bond is moved to between carbon 2 and carbon 3.
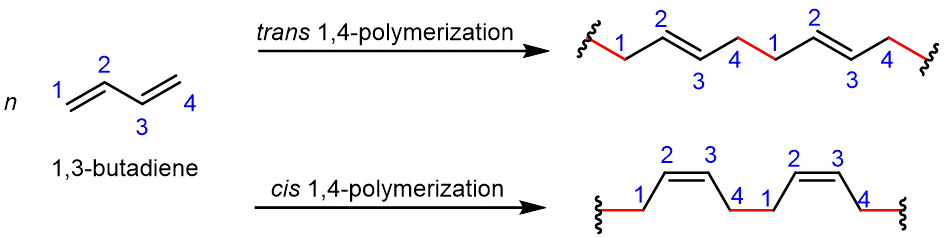
Let’s consider the reaction in a bit more detail. During the polymerization reaction: one electron from the C1=C2 π bond in monomer B pairs with an electron from an adjacent monomer A to form a new σ bond involving C1; similarly, one electron from the C3=C4 π bond in monomer B pairs with an electron from another adjacent monomer C to form a new σ bond involving C4; the other electron from each π bond moves to the center of the molecule, and forms a new π bond between C2 and C3.
Activity 2: 1,4-Addition Polymerization
Chemical reactions involving double bonds on adjacent polymer chains can lead to cross-linking, which enhances elasticity of the polymer. In 1839, Charles Goodyear discovered that when natural rubber was heated to 140–160 °C in the presence of sulfur, the rubber became tougher, more resistant to heat and cold, and more elastic. This process was later called vulcanization after Vulcan, the Roman god of fire and volcanos. Above 140 °C, S–S bonds in sulfur molecules, S8, break, and linear chains of sulfur atoms form. These chains then react with some of the remaining double bonds in the polymer, forming cross links. The development of vulcanized rubber for automobile tires greatly aided the automobile industry.
Another important conjugated diene used in synthetic rubber is chloroprene (2-chloro-1,3-butadiene). Polymerized chloroprene was developed by DuPont and given the trade name Neoprene. Cross-linking in polychloroprene involves combination of two chlorine atoms from adjacent chains with a Zn2+ ion to form ZnCl2. The C–Cl bonds in the uncross-linked polymer become C–C bonds—the cross-link. Cross-linking contributes to the overall elasticity of neoprene.
D14.5 Copolymers
Some of the most commercially important addition polymers are copolymers, made by polymerizing a mixture of two or more monomers. For example, styrene-butadiene rubber (SBR), which is a copolymer of 1,3-butadiene and styrene mixed in about a 3:1 ratio.
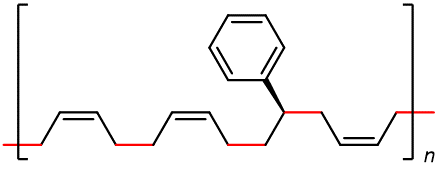
The properties of a copolymer are distinct from those of a mixture of the single polymers. For instance, the properties of SBR are different from a mixture containing polybutadiene and polystyrene, no matter what the ratio of the mixture is.
SBR was developed in the U.S. during World War II when important supplies of natural rubber were cut off. It is more resistant to abrasion and oxidation than natural rubber and can also be vulcanized. More than 40% of the synthetic rubber production is SBR, which is used in tire production. Several other types of rubber are copolymers, such as butyl rubber, which is copolymerized from 2-methylpropene (H2C=C(CH3)2) and a small percentage of isoprene.
Exercise 3: Monomer(s) from Polymer Structure
Day 14 Pre-class Podia Problem: Polymers and Intermolecular Forces
The strength or toughness of a polymeric material depends on strengths of intermolecular forces between polymer chains and several other factors. Assuming other factors are equal, compare the toughness/strength of polyethylene and polyacrylonitrile. Explain clearly which is stronger and why.
Two days before the next whole-class session, this Podia question will become live on Podia, where you can submit your answer.