Additional Reading Materials
Chapter 7: Noncovalent Interactions and Functional Groups
Ch7.1 Forces Between Molecules
Consider these two aspects of the molecular-level environments in solid, liquid, and gaseous matter:
- Particles in a solid are tightly packed together and often arranged in a regular pattern; in a liquid, they are close together with no regular arrangement; in a gas, they are far apart with no regular arrangement.
- Particles in a solid vibrate about fixed positions and do not generally move in relation to one another; in a liquid, they move past each other but remain in essentially constant contact; in a gas, they move independently of one another except when they collide.
The differences in the properties of a solid, liquid, or gas reflect the strengths of the attractive forces between the atoms, molecules, or ions that make up each phase. The phase in which a substance exists depends on the relative extents of its intermolecular forces (IMFs) and the kinetic energies (KE) of its molecules. IMFs are the various forces of attraction that may exist between the molecules of a substance which serve to hold particles close together. The particles’ KE provides the energy required to overcome the attractive forces and thus increase the distance between particles. Figure 1 illustrates how changes in physical state may be induced by changing the temperature, hence, the average KE, of a given substance.
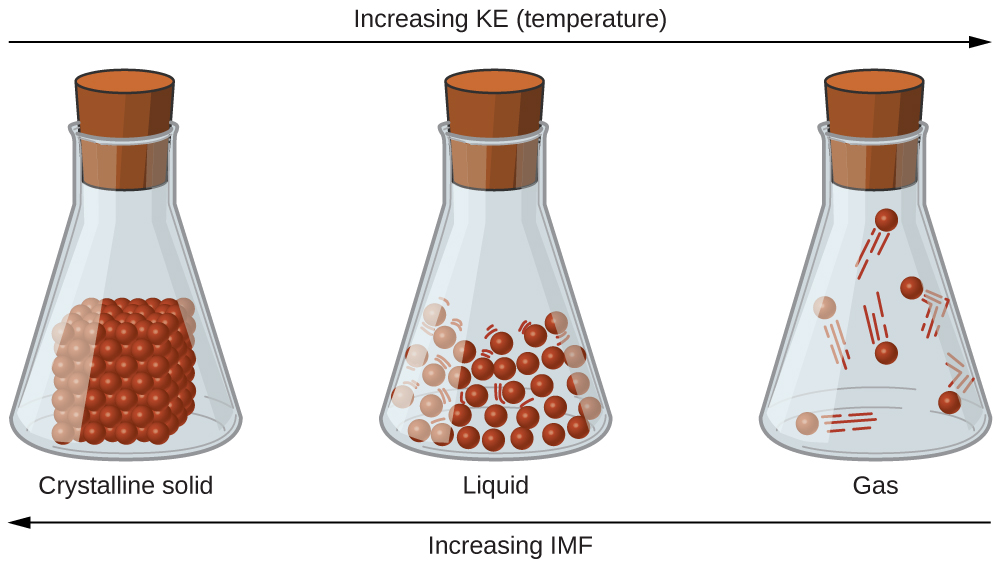
As an example of the processes depicted in this figure, consider a sample of water. When gaseous water is cooled sufficiently, the attractions between H2O molecules will be capable of holding them together when they come into contact with each other; the gas condenses, forming liquid H2O.
Access this interactive simulation on states of matter, phase transitions, and intermolecular forces. This simulation is useful for visualizing concepts introduced throughout this chapter.
Under appropriate conditions, the attractions between all gas molecules will cause them to form liquids or solids. This is due to intermolecular forces, not intramolecular forces. Intramolecular forces are those within the molecule that keep the molecule together, for example, the covalent bonds between the atoms. Intermolecular forces are the attractions between molecules, which determine many of the physical properties of a substance. Figure 2 illustrates these different molecular forces. The strengths of these attractive forces can vary widely, although for mall molecules, the IMFs are usually weaker than intramolecular forces. For example, to overcome the IMFs in liquid HCl and convert it into gaseous HCl requires ~17 kJ/mol. To break the H-Cl covalent bond requires ~25 times more energy—430 kJ/mol.
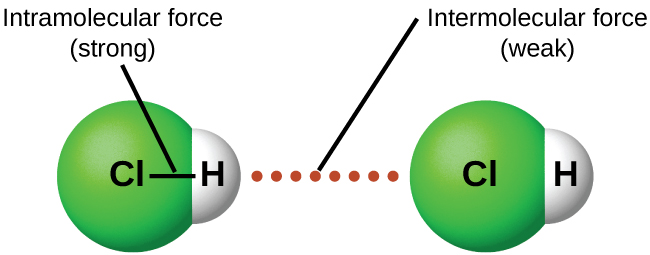
We will often use values such as boiling or enthalpies of vaporization as indicators of the relative strengths of IMFs present within substances, as we explore three major types of IMFs below.
Ch7.2 Dispersion Forces
One of the IMFs that is present in all condensed phases, regardless of the nature of the molecules composing the substance, is called the London dispersion force (in honor of German-born American physicist Fritz London who, in 1928, first explained it). This force is often referred to simply as the dispersion force. Because the electrons of a molecule are in constant motion at any moment in time, a molecule can develop an instantaneous dipole when its electrons are temporarily distributed asymmetrically. The presence of this instantaneous dipole can, in turn, distort the electron distribution of a neighboring molecule, producing an induced dipole. These two rapidly fluctuating, temporary dipoles result in an electrostatic attraction between the molecules—a so-called dispersion force as illustrated in Figure 3.
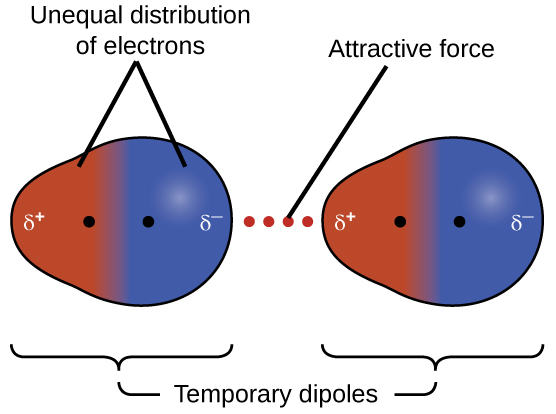
Dispersion forces between molecules can attract the two molecules to each other. However, the forces are weak at long distance, and become significant only when the molecules are very close—it is proportional to [latex]\frac{1}{R^6}[/latex] where R is the intermolecular distance. Molecules with more electrons exhibit stronger dispersion forces than those with less electrons. For example, F2 and Cl2 are gases at room temperature (reflecting weaker attractive forces); Br2 is a liquid, and I2 is a solid (reflecting stronger attractive forces). Trends in observed melting and boiling points for the halogens clearly demonstrate this effect, as seen in Table 1.
Halogen | Number of e– | Atomic Radius | Melting Point | Boiling Point |
---|---|---|---|---|
fluorine, F2 | 18 | 72 pm | 53 K | 85 K |
chlorine, Cl2 | 34 | 99 pm | 172 K | 238 K |
bromine, Br2 | 70 | 114 pm | 266 K | 332 K |
iodine, I2 | 106 | 133 pm | 387 K | 457 K |
astatine, At2 | 170 | 150 pm | 575 K | 610 K |
Table 1. Melting and Boiling Points of the Halogens |
The increase in melting and boiling points with increasing molecular size may be rationalized by considering how the strength of dispersion forces is affected by the electronic structure of the atoms or molecules in the substance. In a larger atom, the valence electrons are, on average, farther from the nuclei than in a smaller atom. Thus, they are less tightly held and can more easily form the temporary dipoles that produce the attraction. The measure of how easy or difficult it is for another electrostatic charge (for example, a nearby ion or polar molecule) to distort a molecule’s charge distribution is known as polarizability. A molecule that has a charge cloud that is more easily distorted is more polarizable and will have larger dispersion forces.
Example 1
London Dispersion Forces and Their Effects
Order the following compounds from lowest to highest boiling point: CH4, SiH4, GeH4, and SnH4. Explain your reasoning.
Solution
All of these molecules have a symmetric tetrahedral molecular geometry, and therefore are nonpolar. These compounds experience only dispersion forces. Hence, the molecule with less electrons will be less polarizable and have weaker dispersion forces. The number of electrons in CH4, SiH4, GeH4, and SnH4 are 10, 18, 36, and 54, respectively. Therefore, the order from lowest to highest boiling point is expected to be CH4 < SiH4 < GeH4 < SnH4.
A graph of the actual boiling points of these compounds versus the period of the group 14 element shows this prediction to be correct:
Check Your Learning
Order the following hydrocarbons from lowest to highest boiling point: ethane (C2H6), propane (C3H8), and n-butane (C4H10).
Answer:
C2H6 < C3H8 < C4H10. All of these compounds are essentially nonpolar and only have London dispersion forces, therefore the molecule with more electrons (here, the longer chain linear alkane) will have stronger dispersion forces and the higher boiling point.
The shapes of molecules also affect the magnitudes of the dispersion forces between them. For example, boiling points for the isomers n-pentane, isopentane, and neopentane (Figure 4) are 36 °C, 27 °C, and 9.5 °C, respectively, even though these molecules have the same chemical formula, C5H12, and therefore same number of electrons. The difference in boiling points suggests that dispersion forces in the liquid phase are greatest for n-pentane and least for neopentane. In n-pentane, the elongated shape provides a greater surface area between n-pentane molecules when they come in contact, resulting in stronger dispersion forces. Neopentane molecules are the most compact of the three, offering the least available surface area for intermolecular contact and, hence, the weakest dispersion forces.
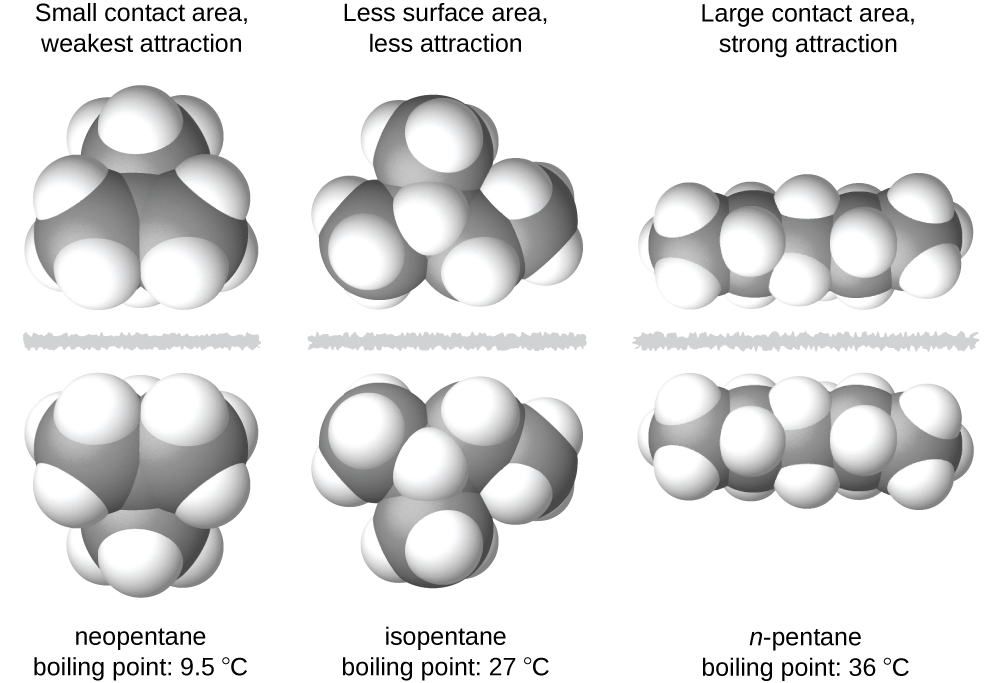
Geckos and Intermolecular Forces
Geckos have an amazing ability to adhere to most surfaces. They can quickly run up smooth walls and across ceilings that have no toe-holds, and they do this without having suction cups or a sticky substance on their toes. And while a gecko can lift its feet easily as it walks along a surface, if you attempt to pick it up, it sticks to the surface. How are geckos (as well as spiders and some other insects) able to do this? Although this phenomenon has been investigated for hundreds of years, scientists only recently uncovered the details of the process that allows geckos’ feet to behave this way.
Geckos’ toes are covered with hundreds of thousands of tiny hairs known as setae, with each seta, in turn, branching into hundreds of tiny, flat, triangular tips called spatulae. The huge numbers of spatulae on its setae provide a gecko with a large total surface area for sticking to a surface. In 2000, Kellar Autumn, who leads a multi-institutional gecko research team, found that geckos adhered equally well to both polar silicon dioxide and nonpolar gallium arsenide. This proved that geckos stick to surfaces because of dispersion forces—intermolecular attractions arising from temporary, synchronized charge distributions between adjacent molecules. The total attraction over millions of spatulae is large enough to support many times the gecko’s weight.
In 2014, two scientists developed a model to explain how geckos can rapidly transition from “sticky” to “non-sticky.” Alex Greaney and Congcong Hu at Oregon State University described how geckos can achieve this by changing the angle between their spatulae and the surface. Geckos’ feet, which are normally nonsticky, become sticky when a small shear force is applied. By curling and uncurling their toes, geckos can alternate between sticking and unsticking from a surface, and thus easily move across it. Further investigations may eventually lead to the development of better adhesives and other applications.
(credit photo: modification of work by “JC*+A!”/Flickr)[/caption]
Watch this video to learn more about Kellar Autumn’s research that determined that dispersion forces are responsible for a gecko’s ability to cling and climb.
Ch7.3 Dipole-Dipole Attractions
Polar molecules have a partial positive charge on one side and a partial negative charge on the other side—a separation of charge called a dipole. The attractive force between the positive end of one dipole and the negative end of another dipole is called a dipole-dipole attraction. Consider a polar molecule such as hydrogen chloride, HCl, where the more electronegative Cl atom bears the partial negative charge and the less electronegative H atom bears the partial positive charge. In addition to dispersion forces, there are also dipole-dipole attraction between HCl molecules, as illustrated in Figure 5.
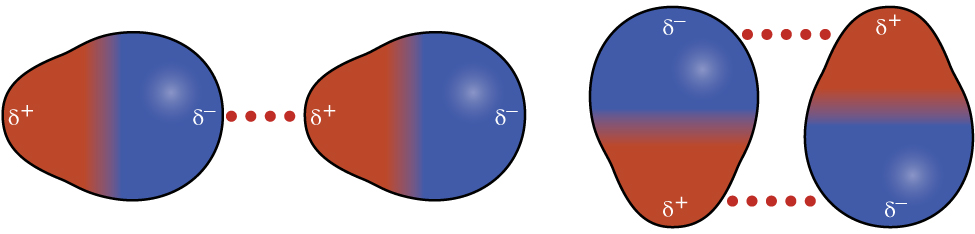
The effect of dipole-dipole attraction is apparent when we compare the properties of HCl to the nonpolar F2. Both HCl and F2 consist of 18 electrons and have approximately the same shape. At a temperature of 150 K, molecules of both substances would have the same average KE. However, HCl is a liquid at that temperature, whereas F2, with only dispersion forces, is gaseous. The higher boiling point of HCl (188 K) compared to F2 (85 K) is a reflection of the additional dipole-dipole attractions between HCl molecules.
The strength of dipole-dipole attractions is dependent on the dipole moment of a molecule, which measures the extent of net charge separation in the molecule as a whole. Dipole moment has a unit of Debye (D). We determine the dipole moment by summing all the individual bond dipole moments while taking into account the 3D molecular structure.
If the bonds in a molecule are arranged such that their bond dipole moments cancel (vector sum equals zero), then the molecule is nonpolar. For example, in the linear CO2 molecule (Figure 6), each of the bonds is polar, but the molecule as a whole is nonpolar. This is because the bond dipole moments of the polar C=O bonds on opposite sides of the carbon atom cancel since they are equal in magnitude and are pointed in opposite directions. Other nonpolar molecules include BF3 (trigonal planar), CH4 (tetrahedral), PF5 (trigonal bipyramidal), and SF6 (octahedral), in which all the polar bonds are identical and are arranged such that their dipoles cancel.
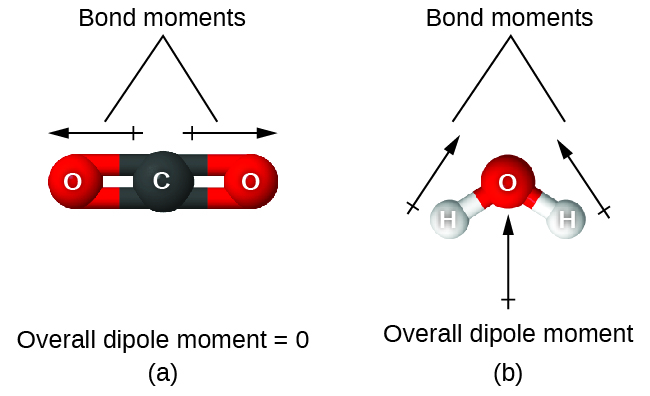
On the other hand, the water molecule (Figure 6) is bent because of the lone pairs on O, and the two O-H bond dipole moments do not cancel. Therefore, a water molecule has a net molecular dipole moment and is a polar molecule.
The OCS molecule has a structure similar to CO2, but a sulfur atom has replaced one of the oxygen atoms. To determine if this molecule is polar, we draw the molecular structure, which is linear:
The C=O bond is considerably polar. Although C and S have very similar electronegativity values, S is slightly more electronegative than C, and so the C=S bond is just slightly polar. Hence OCS is a polar molecule, and because oxygen is more electronegative than sulfur, the oxygen end of the molecule is the negative end.
Chloromethane, CH3Cl, is another example of a polar molecule. Although the polar C–Cl and C–H bonds are arranged in a tetrahedral geometry, the C–Cl bond has a larger bond moment than the C–H bond. Additionally, all of the bond dipole moments have an upward component in the orientation shown, since carbon is more electronegative than hydrogen and less electronegative than chlorine:
Example 2
Polarity Simulations
Open the molecule polarity simulation and select the “Three Atoms” tab at the top. This should display a molecule ABC with three electronegativity adjustors. You can display or hide the bond dipole moments, molecular dipole momentss, and partial charges at the right. Turning on the Electric Field will show whether the molecule moves when exposed to a field.
Use the electronegativity controls to determine how the molecular dipole will look for the starting bent molecule if:
(a) A and C are very electronegative and B is in the middle of the range.
(b) A is very electronegative, and B and C are not.
Solution
(a) Molecular dipole moment points immediately between A and C.
(b) Molecular dipole moment points along the A–B bond, toward A.
Check Your Learning
Determine the partial charges that will give the largest possible bond dipole moments.
Answer:
The largest bond dipole moments will occur with the largest partial charges. The two solutions above represent how unevenly the electrons are shared in the bond. The bond moments will be maximized when the electronegativity difference is greatest. The controls for A and C should be set to one extreme, and B should be set to the opposite extreme. Although the magnitude of the bond dipole moment will not change based on whether B is the most electronegative or the least, the direction of the bond dipole moment will.
Example 3
Dipole-Dipole Forces and Their Effects
Predict which will have the higher boiling point: N2 or CO. Explain your reasoning.
Solution
CO and N2 are both diatomic molecules with 14 electrons, so they experience similar London dispersion forces. Because CO is a polar molecule, it also experiences dipole-dipole attractions. N2 is nonpolar, its molecules cannot exhibit dipole-dipole attractions. The additional dipole-dipole attractions between CO molecules is expected to lead to a higher boiling point. [The boiling point of N2 is 77 K, the boiling point of CO is 82 K.]
Check Your Learning
Predict which will have the higher boiling point: ICl or Br2. Explain your reasoning.
Answer:
ICl. ICl and Br2 have the same number of electrons (70) and therefore experience similar London dispersion forces. ICl is polar and thus also exhibits dipole-dipole attractions; Br2 is nonpolar and does not. The additional dipole-dipole attractions require additional energy to overcome, so ICl will have the higher boiling point.
Ch7.4 Hydrogen Bonding
Nitrosyl fluoride (ONF, 24 electrons, boiling point 201 K) is a gas at room temperature. Water (H2O, 10 electrons, boiling point 373 K) is a liquid. We clearly cannot attribute this difference between the two compounds to dispersion forces, since both molecules have about the same shape and ONF has more electrons. We also cannot attribute it to differences in molecular dipole moments, since both molecules are polar and exhibit comparable dipole moments. This large difference in boiling points is due to the presence of an additional IMF, called hydrogen bonding. Hydrogen bonding occurs between a hydrogen atom that is covalently bonded to F, O, or N (denoted as X) and an electron lone pair on F, O, or N (denoted as Z). These three atoms, F, O, and N, are among the most electronegative elements in the periodic table, as well as being some of the smallest elements. These characteristics give rise to the formation of hydrogen bond (denoted at dotted bond): X-H···Z. Figure 7 illustrates hydrogen bonding between water molecules.
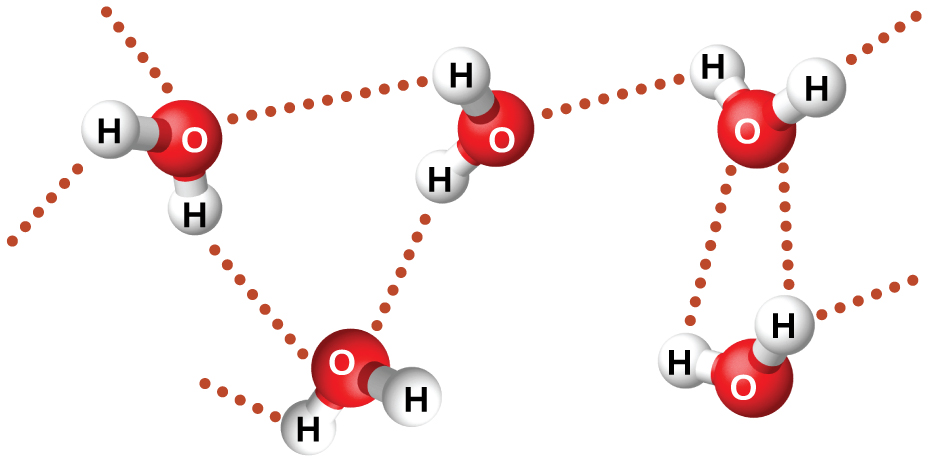
Despite use of the word “bond,” hydrogen bonds are still intermolecular attractive forces. A hydrogen bond is weaker than a typical covalent bond, only about 5-10% as strong, but it is stronger than dispersion forces between small molecules and many dipole-dipole attractions. Hydrogen bond is a distinctive intermolecular force from dipole-dipole attraction—while all substances that exhibit hydrogen bonding are polar molecules, not all polar molecules exhibit hydrogen bonding. In addition, dipole-dipole interactions only accounts for a part of the overall strength found in hydrogen bonding.
For small molecules, hydrogen bonds have a pronounced effect on a substance’s properties in condensed phases (liquids and solids). For example, consider the trends in boiling points for the binary hydrides of group 15 (NH3, PH3, AsH3, and SbH3), group 16 (H2O, H2S, H2Se, and H2Te), and group 17 (HF, HCl, HBr, and HI). The boiling points of the three heavier hydrides for each group are plotted in Figure 8. As we progress down any of these groups, the polarities of the molecules decrease slightly, whereas the number of electrons increase substantially. The effect of increasingly stronger dispersion forces dominates that of slightly weaker dipole-dipole attractions, and the boiling points are observed to increase steadily.
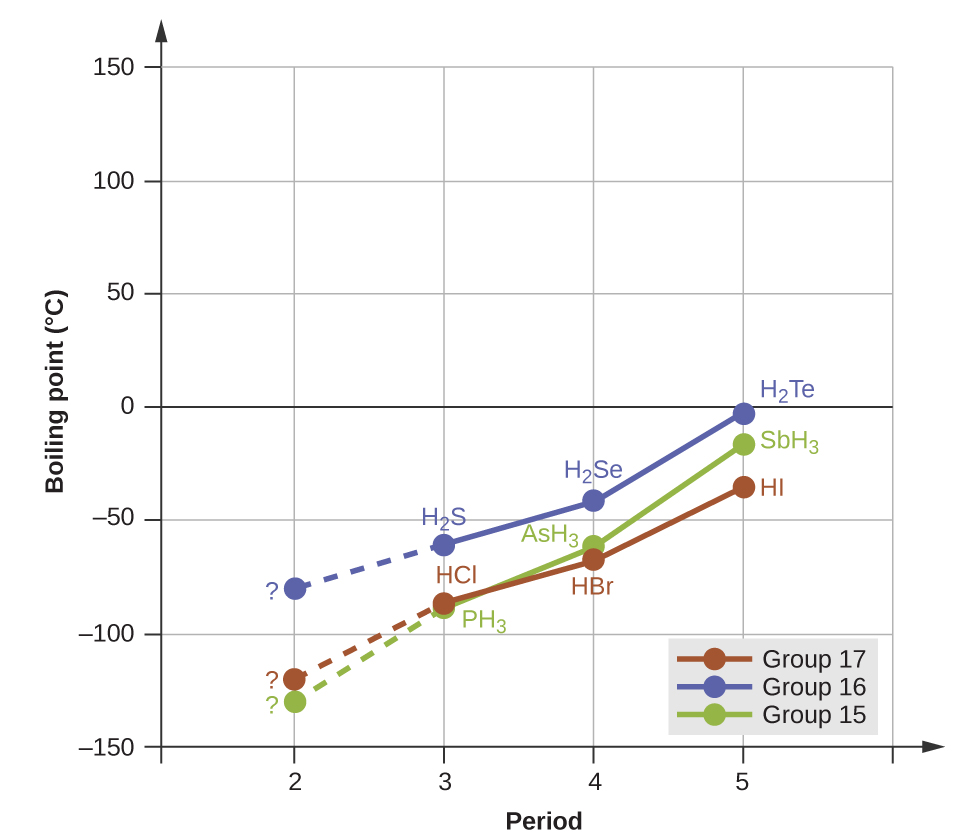
If we use this trend to predict the boiling points for the lightest hydride for each group, we would expect NH3 to boil at about −120 °C, H2O to boil at about −80 °C, and HF to boil at about −110 °C. However, the experimentally meastured boiling points for these compounds are dramatically higher than the trends would predict, as shown in Figure 9. The stark contrast between our predictions and reality provides compelling evidence for the presence of hydrogen bonding.
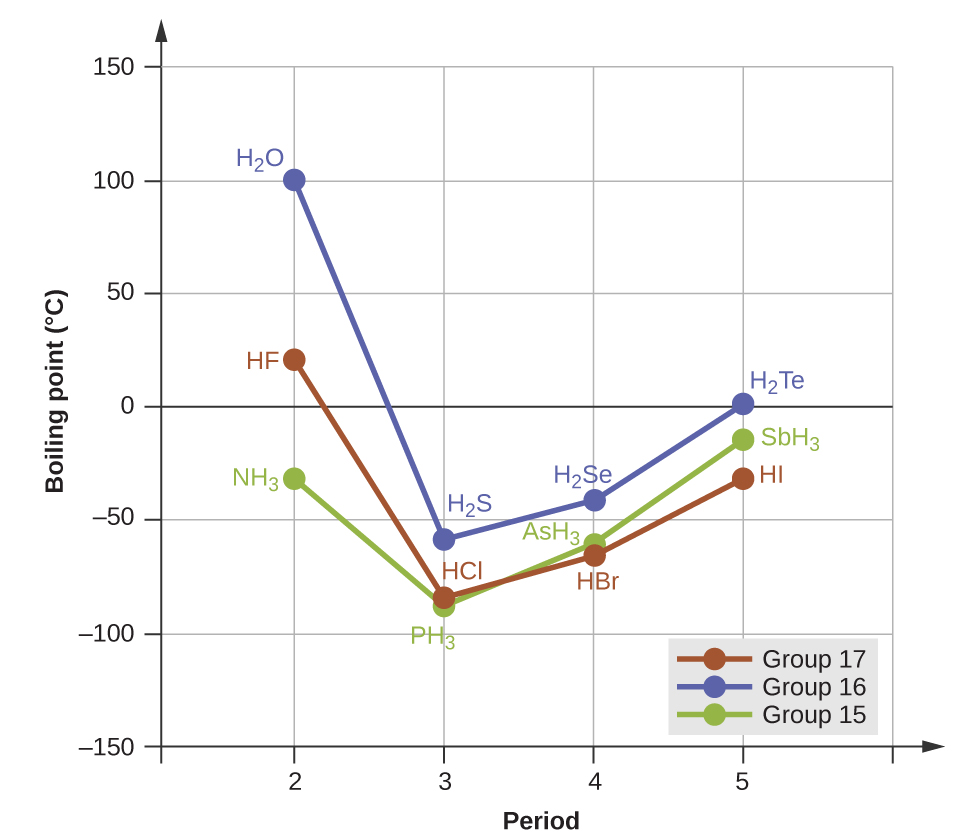
Example 4
Effect of Hydrogen Bonding on Boiling Points
Consider the compounds dimethylether (CH3OCH3), ethanol (CH3CH2OH), and propane (CH3CH2CH3). Their boiling points, not necessarily in order, are −42.1 °C, −24.8 °C, and 78.4 °C. Match each compound with its boiling point. Explain your reasoning.
Solution
The 3D geometry of CH3OCH3, CH3CH2OH, and CH3CH2CH3 are similar, and they all have 26 electrons, so they will exhibit similar dispersion forces. Since CH3CH2CH3 is nonpolar, it exhibit only dispersion forces. Because CH3OCH3 is polar, it will also experience dipole-dipole attractions. Finally, CH3CH2OH has an −OH group, and so it will experience dispersion forces, dipole-dipole attraction and hydrogen bonding. So the ordering in terms of strength of IMFs, and thus boiling points, is CH3CH2CH3 < CH3OCH3 < CH3CH2OH. The boiling point of propane is −42.1 °C, the boiling point of dimethylether is −24.8 °C, and the boiling point of ethanol is 78.4 °C.
Check Your Learning
Would your predict the boiling point of methylamine (CH3NH2) to be greater, smaller, or about the same as ethane (CH3CH3, boiling point −89 °C). Explain your reasoning.
Answer:
The boiling point for methylamine is predicted to be significantly greater than that of ethane. CH3CH3 and CH3NH2 are similar in molecular geometry and number of electrons, but methylamine possesses an −NH group and therefore has dipole-dipole attraction and hydrogen bonding. This greatly increases its IMFs, and therefore its boiling point. (the known boiling point is −6 °C.)
Ch7.5 Alkanes/Petroleum
Each carbon atoms in an alkane has sp3 hybrid orbitals and is bonded to four other atoms, each of which is either carbon or hydrogen. Because of the sp3 hybridization, the bond angles in carbon chains are close to 109.5°, giving such chains in an alkane a zigzag shape.
The number of carbon atoms present in an alkane has no limit. Greater numbers of atoms in the molecules will lead to stronger intermolecular dispersion forces and correspondingly different physical properties. Properties such as melting point and boiling point (Table 2) usually vary smoothly and predictably as a function of the number of carbon and hydrogen atoms in a linear alkane molecule.
Alkane* | Molecular Formula | Melting Point (°C) | Boiling Point (°C) | Phase at STP** | Number of Constitutional Isomers |
---|---|---|---|---|---|
methane | CH4 | –182.5 | –161.5 | gas | 1 |
ethane | C2H6 | –183.3 | –88.6 | gas | 1 |
propane | C3H8 | –187.7 | –42.1 | gas | 1 |
butane | C4H10 | –138.3 | –0.5 | gas | 2 |
pentane | C5H12 | –129.7 | 36.1 | liquid | 3 |
hexane | C6H14 | –95.3 | 68.7 | liquid | 5 |
heptane | C7H16 | –90.6 | 98.4 | liquid | 9 |
octane | C8H18 | –56.8 | 125.7 | liquid | 18 |
nonane | C9H20 | –53.6 | 150.8 | liquid | 35 |
decane | C10H22 | –29.7 | 174.0 | liquid | 75 |
tetradecane | C14H30 | 5.9 | 253.5 | solid | 1858 |
octadecane | C18H38 | 28.2 | 316.1 | solid | 60,523 |
Table 2. Properties of Some Alkanes
*Physical properties for C4H10 and heavier molecules are those of the normal isomer, n-butane, n-pentane, etc. **STP indicates a temperature of 0 °C and a pressure of 1 atm. |
As was discussed earlier, molecular shapes also affect the magnitudes of the dispersion forces. For example, 2-methylpropane (or isobutane) has the same C4H10 molecular formula as n-butane, but has a lower boiling point of -11.7 °C.
Alkanes are relatively stable molecules, but heat or light will activate reactions that involve the breaking of C–H or C–C single bonds. Combustion is one such reaction:
CH4(g) + 2O2(g) → CO2(g) + 2H2O(g)
Alkanes burn in the presence of oxygen, a highly exothermic oxidation-reduction reaction that produces carbon dioxide and water. As a consequence, alkanes are excellent fuels. For example, methane, CH4, is the principal component of natural gas. Butane, C4H10, used in camping stoves and lighters is an alkane. Gasoline is a liquid mixture of continuous- and branched-chain alkanes, each containing from five to nine carbon atoms, plus various additives to improve its performance as a fuel. Kerosene, diesel oil, and fuel oil are primarily mixtures of alkanes with higher molecular masses. The main source of these liquid alkane fuels is crude oil, a complex mixture that is separated by fractional distillation. Fractional distillation takes advantage of the differences in the boiling points of the various components of the mixture (see Figure 10), which arises from the differences in their intermolecular interactions.
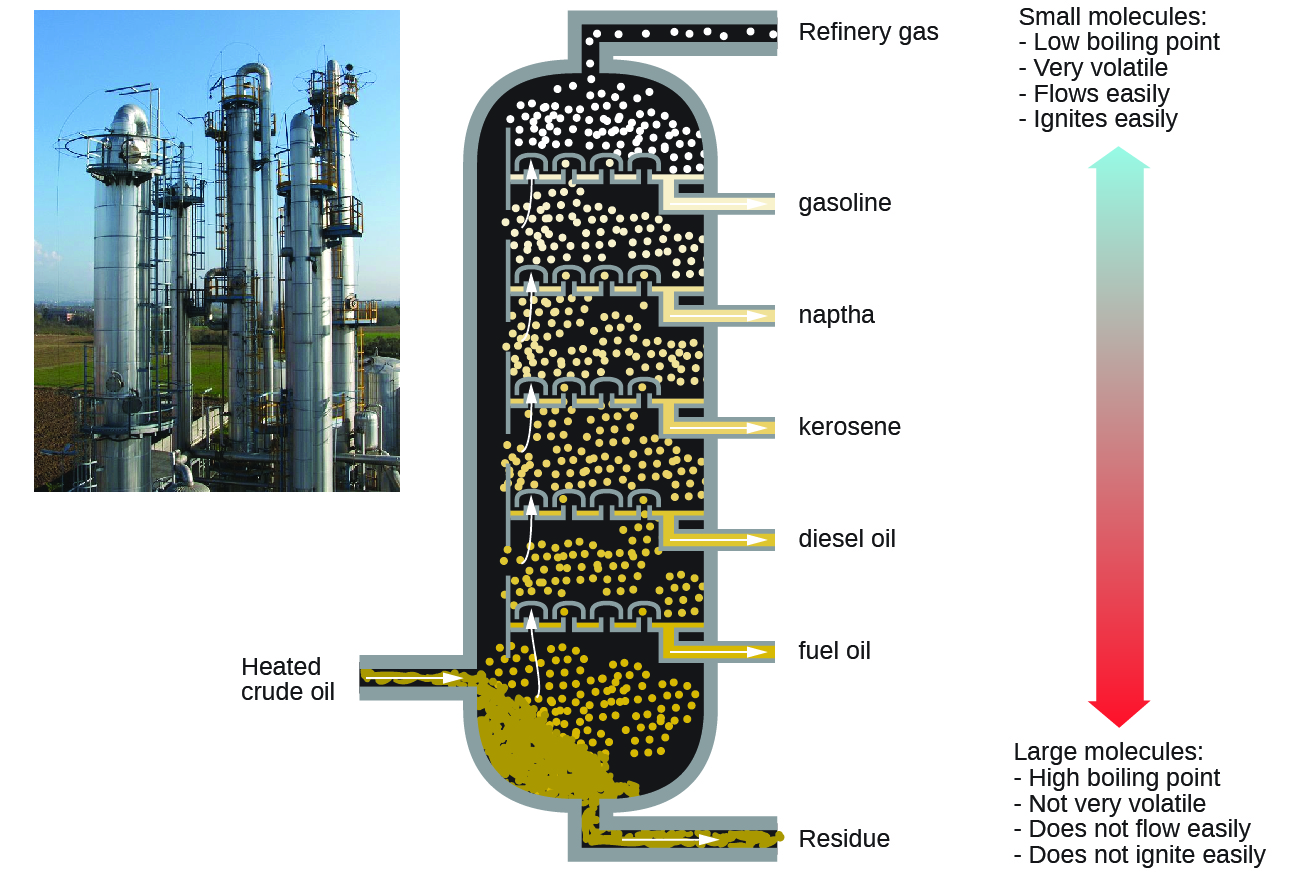
Cracking is the name given to breaking up large hydrocarbon molecules into smaller and more useful bits. This is achieved by using high pressures and temperatures without a catalyst, or lower temperatures and pressures in the presence of a catalyst. The source of the large hydrocarbon molecules is often the naphtha fraction or the gas oil fraction from the fractional distillation of crude oil (petroleum). These fractions are obtained from the distillation process as liquids, but are re-vaporized before cracking.
There is not any single unique reaction happening in the cracker. The hydrocarbon molecules are broken up in a fairly random way to produce mixtures of smaller hydrocarbons, some of which have carbon-carbon double bonds. One possible reaction involving the hydrocarbon C15H32 might be:
Or, showing more clearly what happens to the various atoms and bonds:
This is only one way in which this particular molecule might break up. The ethene and propene are important materials for making plastics or producing other organic chemicals. The octane is one of the molecules found in petrol (gasoline).
Ch7.6 Alcohols
Alcohols are derivatives of hydrocarbons in which an –OH group replaces a hydrogen atom. Although all alcohols have one or more hydroxyl (–OH) functional groups, they do not behave like bases such as NaOH. NaOH is an ionic compound that contains the OH– ions. Alcohols are covalent molecules; the hydroxyl group in an alcohol molecule is covalently bonded to a carbon atom.
Ethanol, CH3CH2OH, also called ethyl alcohol, is an important alcohol for human use. Ethanol is the alcohol produced by some species of yeast, and has long been prepared by humans via harnessing the metabolic efforts of these yeasts in fermenting various sugars:
Large quantities of ethanol can be synthesized from the addition reaction of water with ethylene using an acid as a catalyst:
Alcohols containing two or more hydroxyl groups can be made. Examples include 1,2-ethanediol (ethylene glycol, used in antifreeze) and 1,2,3-propanetriol (glycerine, used as a solvent for cosmetics and medicines):
Ethylene glycol is the principal component of engine coolant for automobiles and is also used to manufacture polyester fibers. In 2005, nearly 1.8 × 1010 kg was produced worldwide. Glycerin is used as a lubricant and in the manufacture of explosives:
When nitroglycerin is mixed with a solid material such as nitrocellulose (which is made by treating cotton or wood pulp with nitric acid), the product is a form of dynamite.
Intermolecular Forces
The presence of the -OH functional group in alcohol makes alcohol molecules polar and capable of hydrogen bonding. For example, the ethylene glycol (1,2-ethanediol) molecule has two -OH groups which can participate in hydrogen bonding, and the glycerin (1,2,3-propanetriol) molecule has three. Both substances have rather high boiling points (198°C for ethylene glycol and 290°C for glycerin) and are syrupy, viscous liquids at room temperature. Their resistance to flowing freely is due to the network of hydrogen bonds that links each molecule to several of its fellows, making it more difficult for them to slide past one another. This highlight again the effect of hydrogen bonding on intermolecular forces and physical properties.
The IMFs present in alcohol are dispersion forces, dipole-dipole interaction, and hydrogen bonding. Such molecules will always have higher boiling points than similarly sized molecules without hydrogen bonding, i.e. more heat is necessary to separate them. For example, ethanol and dimethylether (methoxymethane) both have the same molecular formula, C2H6O.
They have the same number of electrons, and a similar length to the molecule. The dispersion forces and dipole-dipole attractions in each will be much the same. However, ethanol has a hydrogen atom attached directly to an oxygen – and that oxygen still has exactly the same two lone pairs as in a water molecule. Hydrogen bonding can occur between ethanol molecules, although not as effectively as in water. The hydrogen bonding is limited by the fact that there is only one O-H bond in each ethanol molecule.
In methoxymethane, the lone pairs on the oxygen are still there, but the hydrogens aren’t sufficiently δ+ (no O-H bond) for hydrogen bonds to form. The effect due to the presence of additional hydrogen bonding in ethanol is reflected in the boiling points of ethanol and methoxymethane:
ethanol (with hydrogen bonding) | 78.5°C | |
methoxymethane (without hydrogen bonding) | -24.8°C |
It is important to realize that dispersion forces and dipole-dipole interactions are also present in alcohol molecules. For example, all the following molecules contain the same number of electrons, and the first two are much the same length. The higher boiling point of the 1-butanol (butan-1-ol) compared to pentane is due to the additional hydrogen bonding as well as dipole-dipole interactions.
Comparing the two alcohols, both boiling points are high but they are not the same. The boiling point of the isobutanol (2-methylpropan-1-ol) isn’t as high as the butan-1-ol because the branching in the molecule lowers the strength of its dispersion forces.
We know that some liquids mix with each other in all proportions; in other words, they have infinite mutual solubility and are said to be miscible. Liquids that mix with water in all proportions are usually polar substances or substances that form hydrogen bonds. For such liquids, the dipole-dipole interactions and/or hydrogen bonding of the solute molecules with the water solvent molecules are at least as strong as those between molecules in the pure solute or in the pure solvent. Hence, the two kinds of molecules mix easily.
Likewise, nonpolar liquids are miscible with each other because there is no appreciable difference in the strengths of solute-solute, solvent-solvent, and solute-solvent intermolecular attractions. The solubility of polar molecules in polar solvents and of nonpolar molecules in nonpolar solvents is an illustration of the chemical axiom “like dissolves like.”
Two liquids that do not mix to an appreciable extent are called immiscible. Layers are formed when we pour immiscible liquids into the same container. Gasoline, benzene, carbon tetrachloride, and many other nonpolar liquids are immiscible with water. The attraction between the molecules of such nonpolar liquids and polar water molecules is ineffectively weak. The only strong attractions in such a mixture are between the water molecules, so they effectively squeeze out the molecules of the nonpolar liquid. The distinction between immiscibility and miscibility is really one of degrees, such that miscible liquids are of infinite mutual solubility, while liquids said to be immiscible are of very low (though not zero) mutual solubility.
Two liquids, such as bromine and water, that are of moderate mutual solubility are said to be partially miscible. Two partially miscible liquids usually form two layers when mixed. In the case of the bromine and water mixture, the upper layer is water, saturated with bromine, and the lower layer is bromine saturated with water. Since bromine is nonpolar, and, thus, not very soluble in water, the water layer is only slightly discolored by the bright orange bromine dissolved in it. Since the solubility of water in bromine is very low, there is no noticeable effect on the dark color of the bromine layer (Figure 11).
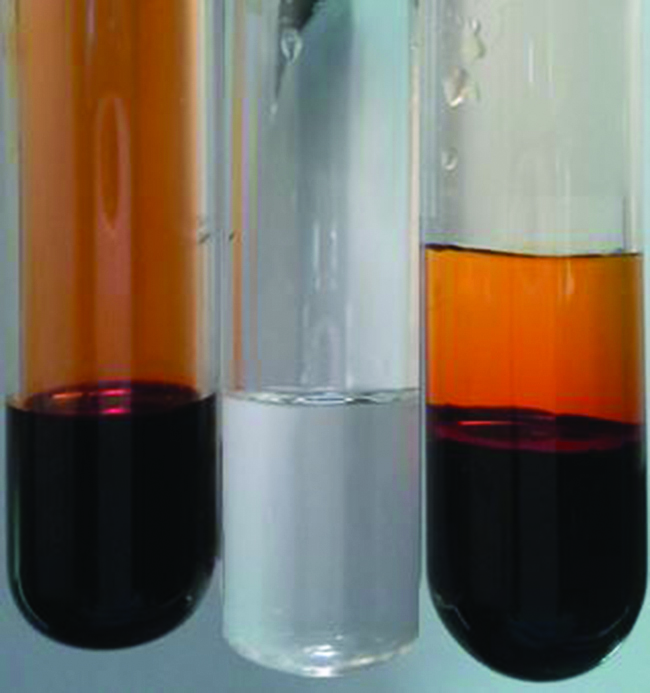
For alcohol molecules, the -OH end is polar and capable of hydrogen bonding, making it hydrophilic (“water-loving”), capable of strong intermolecular interactions with water molecules. On the other hand, the hydrocarbon (alkyl) end of an alcohol molecule is nonpolar and is hydrophobic (“water-fearing”), where the only interaction with water molecules are dispersion forces. Hence, although the alkyl part of the molecule does not influence the reactivity of alcohol molecules, it does have a significant impact on the solubility of alcohols in water. Alcohols with small alkyl groups, e.g. methanol and ethanol, are completely miscible in water, while larger alcohol molecules, such as 1-octanol, are immiscible in water.
Oxidation Reactions
A carbon atom typically forms four bonds. Therefore, in an alcohol where carbon is bonded to an -OH group, there can be up to three carbon atoms directly bonded to the carbon atom bonded to the oxygen in -OH. As such, alcohols can be classified according to the number of alkyl groups attached to the carbon with the hydroxyl group. If one alkyl group is attached to that carbon, the alcohol is a primary (1º) alcohol. If two alkyl groups are attached, the alcohol is a secondary (2º) alcohol. If three alkyl groups are attached, the alcohol is a tertiary (3º) alcohol (Figure 12).
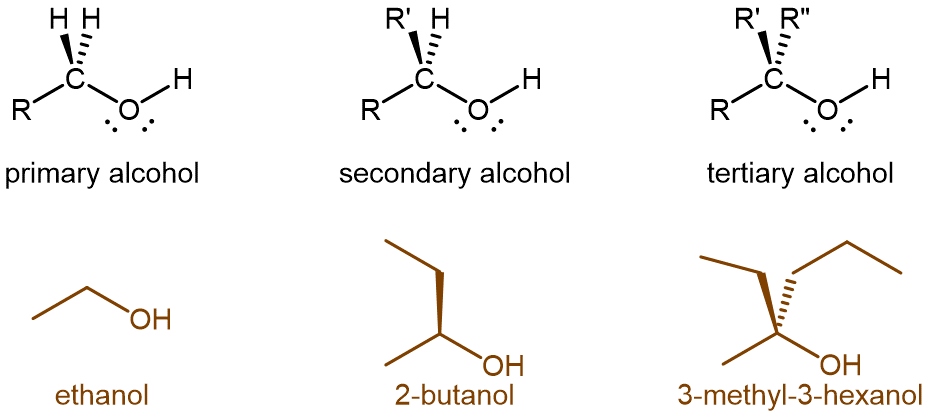
All alcohols can be completely oxidized to carbon dioxide and water by oxygen in the air; that is, all alcohols are combustible. Like hydrocarbons, combustion is an important reaction of alcohols, but controlled oxidation is even more important because it can convert alcohols into other compounds that are chemically useful. The ease with which an alcohol can be oxidized and the extent of the oxidation depends on whether the alcohol is primary, secondary, or tertiary.
For primary alcohols, controlled, stepwise oxidation first yields compounds called aldehydes, then aldehydes can be further oxidized to carboxylic acids.
Oxidation of an organic compound can usually be recognized because either an oxygen atom is added to a molecule or two hydrogen atoms are lost from a molecule. For example, stepwise oxidation of ethanol first produces the aldehyde ethanal (commonly called acetaldehyde); further oxidation produces the carboxylic acid, ethanoic acid (commonly called acetic acid). An aldehyde has the functional group –CHO, where the carbon atom is double-bonded to an oxygen atom. A carboxylic acid has the functional group –COOH, in which the carbon atom is double-bonded to an oxygen atom and single-bonded to an oxygen atom in an OH group.
Note that acetaldehyde differs from ethanol by loss of one H atom from the oxygen atom and one H atom from the carbon on the right. Acetic acid differs from acetaldehyde by having an addition O atom on the right-hand carbon atom.
Controlled oxidation can be carried out in the laboratory using an aqueous solution of potassium permanganate (KMnO4(aq)) or an aqueous solution of potassium dichromate (K2Cr2O7(aq)).
When a similar controlled oxidation is applied to a secondary alcohol, such as 2-propanol, the oxidized molecule contains a C=O group that has two other carbon atoms attached to the C atom, this functional group is called a ketone. The ketone formed from 2-propanol is called propanone (commonly called acetone).
Again, note that in the ketone the number of hydrogen atoms is fewer by two than the number in the secondary alcohol. The oxidation corresponds with loss of two hydrogen atoms. Ketones are difficult to oxidize further, because there is no way to add another oxygen atom to the carbon atom in the C=O group, nor is there a way to remove hydrogen atoms from the C and O atoms in the C=O group.
Tertiary alcohols, which have no hydrogen atoms attached to the carbon that is bonded to the –OH group, are difficult to oxidize. If a primary alcohol, a secondary alcohol, and a tertiary alcohol are dissolved in water in three beakers and then treated with either potassium permanganate or potassium dichromate, only the primary and secondary alcohols will react. (The reaction can be observed because both permanganate ions and dichromate ions are colored (purple and orange, respectively). Thus for the primary and secondary alcohols, the color will disappear, but for tertiary alcohols there will be no color change.
Ch7.7 Carboxylic Acids
The odor of vinegar is caused by the presence of acetic acid, a carboxylic acid, in the vinegar. Carboxylic acids contain a carbonyl group (C=O) and a second oxygen atom bonded to the carbon atom in the carbonyl group by a single bond. The second oxygen atom also bonds to a hydrogen atom. The names for carboxylic acids include prefixes that denote the lengths of the carbon chains in the molecules and are derived following nomenclature rules similar to those for inorganic acids and salts (The functional group for a carboxylic acid is shown in red on the left):
The simplest carboxylic acid is formic acid, HCOOH, known since 1670. Its name comes from the Latin word formicus, which means “ant”; it was first isolated by the distillation of red ants. It is partially responsible for the pain and irritation of ant and wasp stings, and is responsible for a characteristic odor of ants that can be sometimes detected in their nests.
Acetic acid, CH3COOH, constitutes 3–6% of vinegar. Cider vinegar is produced by allowing apple juice to ferment without oxygen present. Yeast cells present in the juice carry out the fermentation reactions, which change the sugar present in the juice to ethanol, then to acetic acid. Pure acetic acid has a penetrating odor and produces painful burns. It is an excellent solvent for many organic and some inorganic compounds, and it is essential in the production of cellulose acetate, a component of many synthetic fibers such as rayon.
Butyric acid, CH3CH2CH2-COOH, a component of rancid butter and Limburger cheese, has a vile odor. Adipic acid (IUPAC name: hexanedioic acid) is an example of a dicarboxylic acid—it has two -COOH functional groups, one on each end—and is used to make nylon.
Since the carboxyl group contains a highly polar C=O as well as an O-H group, hydrogen bonding is extensive among molecules of the carboxylic acids. Pure acetic acid is called glacial acetic acid because its melting point of 16.6 °C is high enough that it can freeze in a cold laboratory. It is also quite thick and syrupy because of extensive hydrogen bonding. The hydrogen bonding interaction strength between carboxylic acid molecules are generally stronger than that between alcohol molecules. For example, the boiling point of acetic acid is 118 °C, while that of 1-propanol is 97 °C and 2-propanol (isopropyl alcohol) is 82.6 °C.
The hydrogen atom in the -COOH group is acidic and will react with a base to form an ionic salt:
Carboxylic acids are weak acids, meaning they are not 100% ionized in water. For example, about 1% of the acetic acid molecules dissolved in water are ionized at any given time. The remaining molecules are undissociated in solution. However, when we look at comparable alcohols, such as ethanol, carboxylic acids are stronger acids by over ten powers of ten! Why should the presence of a carbonyl group adjacent to a hydroxyl group have such a profound effect on the acidity of the hydroxyl proton?
The acidity of a molecule is related to its behavior in acid-base equilibrium (we will learn this in much more detail later on in the semester), and equilibrium favors the thermodynamically more stable side. Consequently, anything that stabilizes the conjugate base (A–) of an acid will make that acid (H–A) more easily dissociate in water (i.e. a stronger acid).
The conjugate base of carboxylic acid, the carboxylate anion, is stabilized by resonance, as shown in Figure 13. The two contributing structures have equal weight in the hybrid, and the C–O bonds are of equal length (between a double and a single bond). Hence, the presence of carbonyl group make this stabilization possible, leading to a markedly increased acidity. In contrast, there’s no such resonance stabilization for the conjugate base of alcohol, the alkoxide anion.
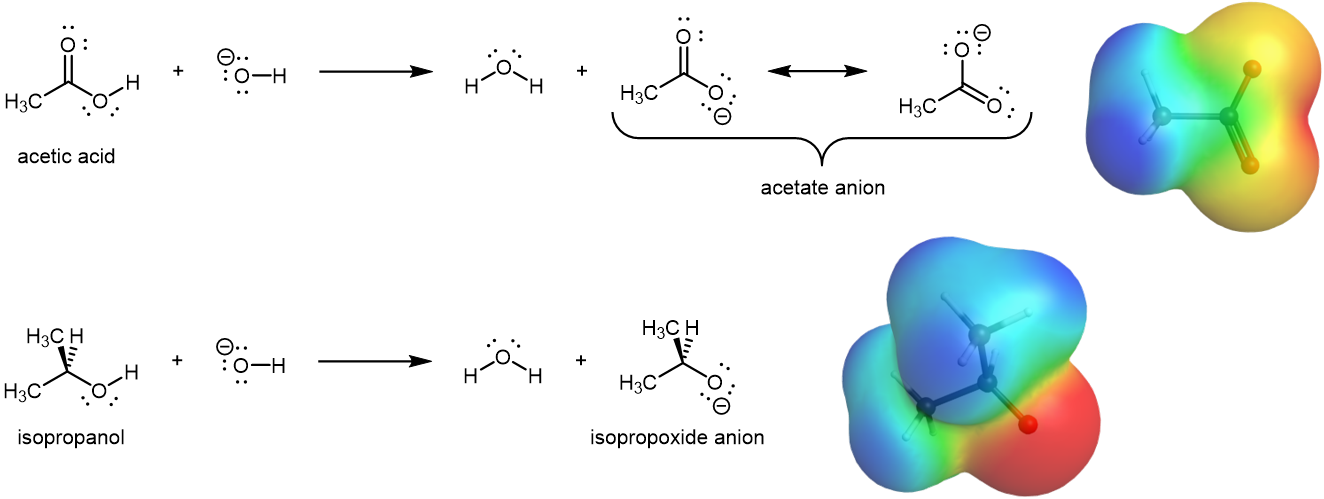
Ch7.8 Esters
The odor of ripe bananas and many other fruits is due to the presence of esters, compounds that can be prepared by the reaction of a carboxylic acid with an alcohol. Because esters do not have hydrogen bonds between molecules, they have lower vapor pressures than the alcohols and carboxylic acids from which they are derived (see Figure 14).
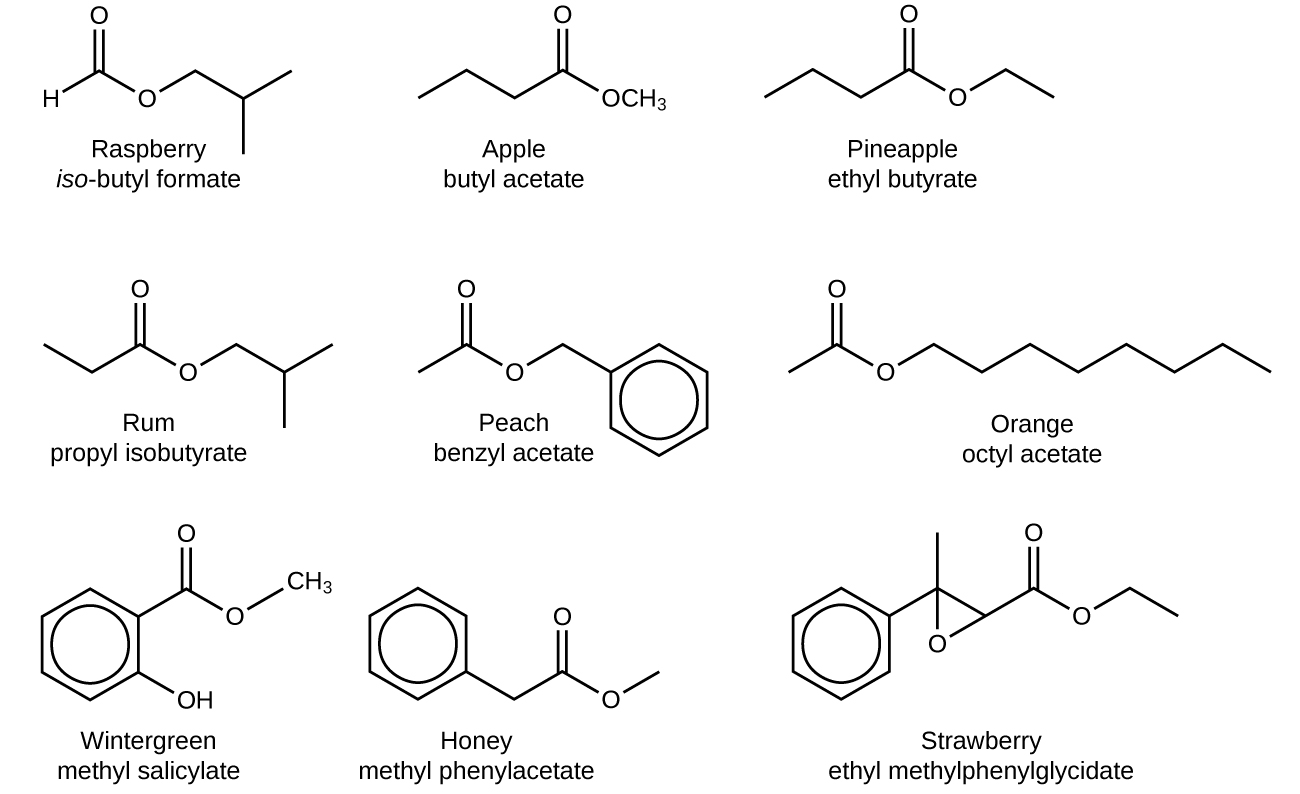
Similar to carboxylic acids, esters contain a carbonyl group (C=O) and a second oxygen atom bonded to the carbonyl carbon. In an ester, the second oxygen atom bonds to another carbon atom. The names for esters include prefixes that denote the lengths of the carbon chains in the molecules (the functional groups for an ester are shown in red on the right):
Esters are produced by the reaction of carboxylic acids with alcohols. For example, ethyl acetate, CH3COOCH2CH3, is formed when acetic acid reacts with ethanol:
The distinctive and attractive odors and flavors of many flowers, perfumes, and ripe fruits are due to the presence of one or more esters (Figure 15). Among the most important natural esters are fats (such as lard, tallow, and butter) and oils (such as linseed, cottonseed, and olive oils), which are esters of the trihydroxyl alcohol glycerine, C3H5(OH)3, with large carboxylic acids, such as palmitic acid, CH3(CH2)14COOH, stearic acid, CH3(CH2)16COOH, and oleic acid, CH3(CH2)7CH=CH(CH2)7COOH . Oleic acid is an unsaturated acid; it contains a C=C double bond. Palmitic and stearic acids are saturated acids that contain no double or triple bonds.
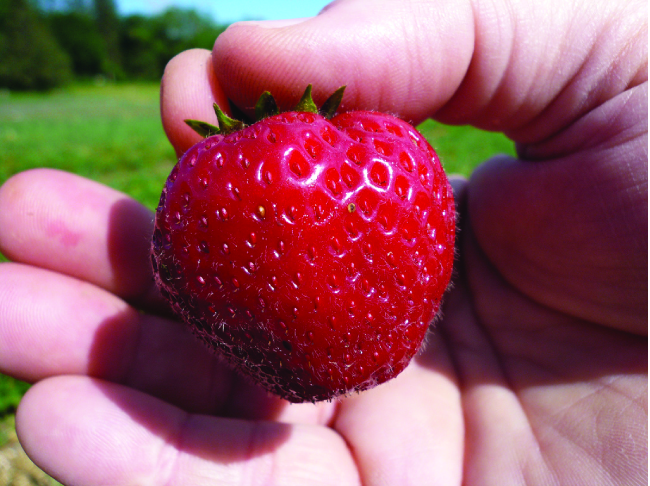
Ch7.9 Amines
Amines are molecules that contain carbon-nitrogen bonds. The nitrogen atom in an amine has a lone pair of electrons and three single bonds to other atoms, either carbon or hydrogen. Various nomenclatures are used to derive names for amines, but all involve the class-identifying suffix –ine as illustrated here for a few simple examples:
Similar to alcohols, you can group amines by the number of carbon atoms bonded to the nitrogen. In the above example, methyl amine is a primary amine, dimethyl amine is a secondary amine, and trimethyl amine is a tertiary amine.
Like ammonia, amines are weak bases due to the lone pair of electrons on their nitrogen atoms:
The basicity of an amine’s nitrogen atom plays an important role in much of the compound’s chemistry. Amine functional groups are found in a wide variety of compounds, including natural and synthetic dyes, polymers, vitamins, and medications such as penicillin and codeine. They are also found in many molecules essential to life, such as amino acids, hormones, neurotransmitters, and DNA.
Addictive Alkaloids
Since ancient times, plants have been used for medicinal purposes. One class of substances, called alkaloids, found in many of these plants has been isolated and found to contain cyclic molecules with an amine functional group. These amines are bases. They can react with H3O+ in a dilute acid to form an ammonium salt, and this property is used to extract them from the plant:
R3N + H3O+ + Cl– → [R3NH+]Cl– + H2O
The name alkaloid means “like an alkali.” Thus, an alkaloid reacts with acid. The free compound can be recovered after extraction by reaction with a base:
[R3NH+]Cl– + OH– → R3N + H2O + Cl–
The structures of many naturally occurring alkaloids have profound physiological and psychotropic effects in humans. Examples of these drugs include nicotine, morphine, codeine, and heroin. The plant produces these substances, collectively called secondary plant compounds, as chemical defenses against the numerous pests that attempt to feed on the plant:
In these diagrams, as is common in representing structures of large organic compounds, carbon atoms in the rings and the hydrogen atoms bonded to them have been omitted for clarity. The solid wedges indicate bonds that extend out of the page. The dashed wedges indicate bonds that extend into the page. Notice that small changes to a part of the molecule change the properties of morphine, codeine, and heroin. Morphine, a strong narcotic used to relieve pain, contains two hydroxyl functional groups, located at the bottom of the molecule in this structural formula. Changing one of these hydroxyl groups to a methyl ether group forms codeine, a less potent drug used as a local anesthetic. If both hydroxyl groups are converted to esters of acetic acid, the powerfully addictive drug heroin results (Figure 16).
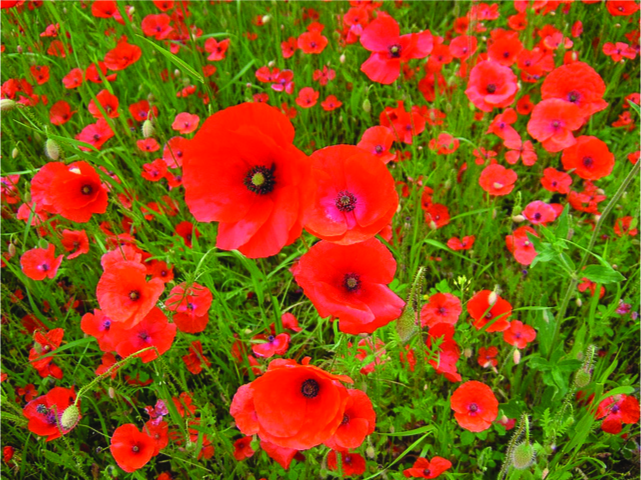
Ch7.10 Amides
Amides are molecules that contain nitrogen atoms connected to the carbon atom of a carbonyl group. Like amines, various nomenclature rules may be used to name amides, but all include use of the class-specific suffix -amide:
Amides can be produced when carboxylic acids react with amines or ammonia. A water molecule is eliminated from the reaction, and the C-N amide bond is formed from the remaining pieces of the carboxylic acid and the amine (note the similarity to formation of an ester from a carboxylic acid and an alcohol):
The reaction between amines and carboxylic acids to form amides is biologically important. It is through this reaction that amino acids (molecules containing both an amine and a carboxylic acid functional group) link together in a polymer to form peptides and proteins.