Unit Four
Day 42: Electrolysis
As you work through this section, if you find that you need a bit more background material to help you understand the topics at hand, you can consult “Chemistry: The Molecular Science” (5th ed. Moore and Stanitski) Chapter 17-10 through 17-11, and/or Chapter 17.11-17.14 in the Additional Reading Materials section.
D42.1 Fuel Cells
Suppose a voltaic cell was constructed so that the substance that was oxidized at the anode and the substance that was reduced at the cathode (the reactants in the overall cell reaction) were both supplied continuously. The battery would never run down because reactant concentrations or partial pressures would never decrease. Such a device is a fuel cell. A fuel cell produces electricity as long as fuel is available. Hydrogen fuel cells have been used to supply power for satellites, space capsules, automobiles, boats, and submarines (Figure 1).
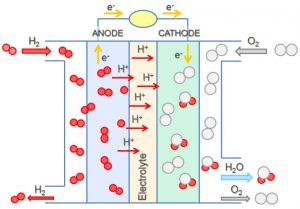
In a hydrogen-oxygen proton-exchangefuel cell, the reactions are:
The cell potential is about 1 V.
The efficiency of fuel cells is typically about 40-60%, which is higher than the typical internal combustion engine (25-35%) and, in the case of the hydrogen fuel cell, produces only water as exhaust. Currently, fuel cells are rather expensive and contain features that may cause a higher failure rate.
D42.2 Electrolysis
In an electrolytic cell electrical energy causes a nonspontaneous reaction to occur in a process known as electrolysis. An electrolytic cell is the opposite of a voltaic cell, where a spontaneous chemical reaction produces electrical energy. Charging a rechargeable battery is an example of electrolysis: electrical energy causes a chemical reaction in the battery, replenishing substances that reacted away as the battery discharged (produced electricity).
For example, consider electrolysis of molten sodium chloride. A simplified diagram of the electrolytic cell used for commercial manufacture of sodium metal and chlorine gas is shown in Figure 2. An external source of direct electric current forces electrons to move into the electrode in the cathode half-cell compartment. The electrons cause reduction of sodium ions to sodium atoms (reduction occurs at the cathode). In the molten salt, sodium ions move toward the cathode and chloride ions move toward the anode (note that negative ions move through the circuit in the same direction as electrons). In the anode half-cell, each chloride ion loses one electron to the electrode surface and oxidation to chlorine gas takes place (oxidation occurs at the anode). The electrons are conducted through a wire to the the voltage source, completing the electric circuit. A porous screen allows movement of ions but not mixing of sodium metal and chlorine gas, which would react spontaneously upon contact.
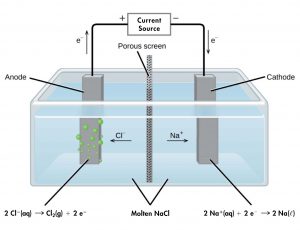
The reactions are:
The negative [latex]E_{\text{cell}}^{\circ}[/latex] indicates that this reaction is strongly reactant-favored, and that, for standard-state conditions, the power supply (battery) must provide at least 4 V to cause the electrolysis reaction to occur. In practice, the applied voltages are higher because of inefficiencies in the process itself and to increase current flow.
In another example, electrolysis can split water into hydrogen gas and oxygen gas. For current to pass through the solution there must be ions present. Acid is typically added to increase the concentration of ions in solution (Figure 3).
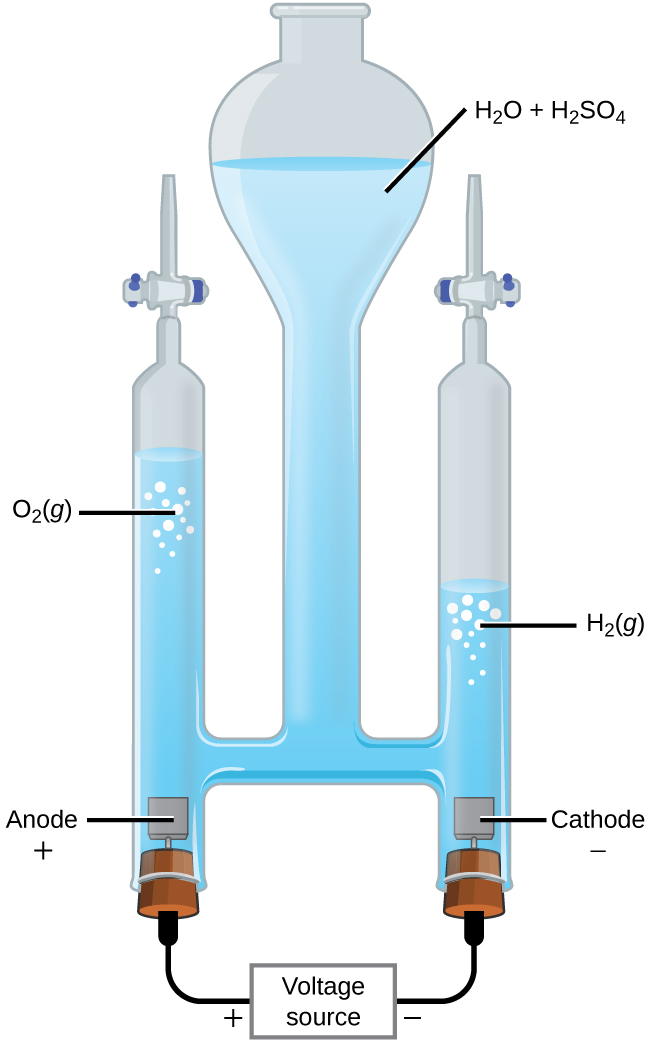
In 1-M acidic solution the reactions and potentials are
At least 1.229 V is required to make this reactant-favored process occur in 1-M H+(aq).
Finally, let’s consider what occurs during the electrolysis of 1-M aqueous potassium iodide. Present in the solution are water molecules, potassium ions, and iodide ions. This example differs from the previous examples because more than one species can be oxidized and more than one species can be reduced. Considering the anode first, the possible oxidation reactions are:
(Oxidation of K+ to K2+ is not considered because K+ has a noble-gas electron configuration and therefore a very high ionization energy.) Because [latex]E_{\text{cell}}^{\circ}[/latex] = [latex]E_{\text{cathode}}^{\circ}[/latex] − [latex]E_{\text{anode}}^{\circ}[/latex], the more positive the anode half-cell potential is, the more negative the cell potential is. Therefore, iodide should be oxidized at the anode because it has a less positive half-cell potential. However, the pH of 1-M KI solution is 7, so reaction (ii) is not at standard-state conditions. The Nernst equation, can be applied to half-cell reactions as well as overall cell reactions. We can assume a temperature of 25 °C and that O2 would be produced at 1 bar, so for reaction (ii)
[latex]\begin{array}{l} E = {E^ \circ } - \dfrac{{RT}}{{nF}}\ln \left( {\dfrac{1}{{{{{\rm{[}}{{\rm{H}}^{\rm{ + }}}{\rm{]}}}^{\rm{4}}}}}} \right) = 1.229{\rm{ V}} - \dfrac{{8.414 \times 298.15}}{{4 \times 96485}}\;{\rm{V}} \times \ln \left( {\dfrac{1}{{{{(1 \times {{10}^{ - 7}})}^4}}}} \right)\\[0.3em] {\rm{ }} = 1.229\;{\rm{ V}} - 0.414\;{\rm{ V}} = 0.815\;{\rm{ V}} \end{array}[/latex]
Eanode = 0.815 V for reaction (ii) at pH = 7. This is still higher than for reaction (i), so reaction (i) occurs and I2 forms at the anode.
Now consider the possible reactions at the cathode:
(Reduction of chloride ion is not considered because Cl− has a noble-gas electron configuration and it is difficult to add more electrons.) Reduction of water, reaction (iii) with Ecathode = -0.4136 V at pH = 7 (calculated for nonstandard conditions using the Nernst equation), is much more likely to occur than is reduction of K+(aq) to K(s) with Ecathode = -2.925 V. (This conclusion is supported by the fact that potassium metal reacts vigorously with water to generate K+(aq), hydrogen gas, and hydroxide ions, so if K(s) formed it would immediately react with water.)
The overall reaction is then:
Electroplating
An important use for electrolytic cells is electroplating, which results in a thin coating of one metal on top of a conducting surface. The metals commonly used in electroplating include cadmium, chromium, copper, gold, nickel, silver, and tin. We can get an idea of how this works by investigating how silver-plated tableware is produced (Figure 5).
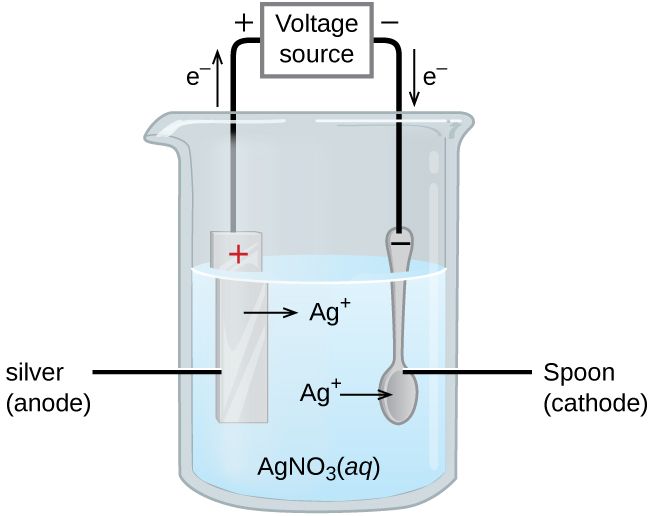
The anode consists of a silver electrode. The cathode is a spoon made from an inexpensive metal. Both electrodes are immersed in a solution of silver nitrate. As the potential is increased, current flows. Silver metal is lost at the anode as it goes into solution:
The mass of the cathode increases as silver ions from the solution are deposited onto the spoon:
The net result is the transfer of silver metal from the anode to the cathode. The quality of the electroplated object depends on the thickness of the deposited silver and the rate of deposition.
D42.3 Quantitative Aspects of Electrolysis
The quantity of current that is allowed to flow in an electrolytic cell is related to the amount (mol) of electrons, which is related to quantities of reactants and products using reaction stoichiometry. Recall that the SI unit for current (I) is the ampere (1 A = 1 C/s). The total charge (Q, in coulombs) is therefore related to current and the time the current flows. It is also related to the amount of electrons (n):
where F is the Faraday constant.
Example 1
Determining Amount of Electrons from Current and Time
In one process used for electroplating silver, a current of 10.23 A was passed through an electrolytic cell for exactly 1 hour. Calculate how many moles of electrons passed through the cell. What mass of silver was deposited at the cathode from the silver nitrate solution?
Solution
Faraday’s constant can be used to convert the charge (Q) into moles of electrons (n). The charge is the current (I) multiplied by the time:
The electroplating solution contains AgNO3, so the reaction at the cathode involves 1 mol electrons for each 1 mol silver:
The atomic mass of silver is 107.9 g/mol, so:
Check your answer: From the stoichiometry, 1 mol electrons would produce 1 mol silver. Less than one-half mol electrons was involved and less than one-half mol silver was produced.
Check Your Learning
Aluminum metal can be made by electrolysis from bauxite ore, which contains aluminum ions . Write the half-reaction at the cathode. Determine the mass of aluminum metal produced if a current of 2.50 × 103 A passed through the solution for 15.0 minutes? Assume the yield is 100%.
Answer:
[latex]\text{Al}^{3+}(\text{aq})\;+\;3\text{e}^{-}\;{\longrightarrow}\;\text{Al}(\text{s})[/latex]; 7.77 mol Al; 210. g Al.
Podia Question
To prepare part of a chromium-plated sculpture, a 0.010-mm thick layer of chromium must be deposited on a part with a total surface area of 3.3 m2 from a solution containing chromium(III) ions. How long would it take to deposit the layer of chromium if the current is 33.46 A? The density of chromium (metal) is 7.19 g/cm3.
Two days before the next whole-class session, this Podia question will become live on Podia, where you can submit your answer.