Unit Two
Day 15: Functional Groups, Addition Polymers
As you work through this section, if you find that you need a bit more background material to help you understand the topics at hand, you can consult “Chemistry: The Molecular Science” (5th ed. Moore and Stanitski) Chapter 10-5 through 10-6a, and/or Chapter 7.7-7.8 and Chapter 8.1 in the Additional Reading Materials section.
D15.1 Carboxylic Acids
Carboxylic acids contain a -COOH functional group, which has a carbonyl and a hydroxyl group linked to the same carbon atom, as shown in red for acetic acid below. A general formula for a carboxylic acid is R–COOH.
The simplest carboxylic acid is formic acid, HCOOH, with R = H, known since 1670. Its name comes from the Latin word for ant, formicus. Formic acid is partially responsible for the pain and irritation of ant and wasp stings. Acetic acid, CH3COOH, the Lewis structure above, is a main component (>4% by volume) of vinegar. Cider vinegar is produced by allowing apple juice to ferment without oxygen present; yeast changes sugar to ethanol, which is then converted to acetic acid via biological oxidation of the primary alcohol. Butyric acid, CH3CH2CH2COOH, a component of rancid butter and Limburger cheese, has a vile odor. Adipic acid (IUPAC name: hexanedioic acid) is an example of a dicarboxylic acid—it has two -COOH functional groups, one on each end. Adipic acid is used to make one type of nylon.
Carboxylic acid molecules are polar and can form hydrogen bonds. Pure acetic acid is called glacial acetic acid because its melting point of 16.6 °C is high enough that it can freeze in a cold laboratory. It is also quite thick and syrupy because of extensive hydrogen bonding. The effect of hydrogen bonding between carboxylic acid molecules is usually greater than between alcohol molecules. For example, the boiling point of acetic acid (32 electrons in the molecule) is 118 °C, while that of 1-propanol (34 electrons) is 97 °C and 2-propanol (isopropyl alcohol, 34 electrons) is 82.6 °C.
Carboxylic acids are acidic because their molecules can donate the hydrogen atom (a proton, H+) in the -COOH group to another molecule or ion. This requires breaking the O–H bond in the -COOH group. Alcohols are not typically thought of as acids, but they are very, very weak acids. Both carboxylic acids and alcohols have –OH in their functional group, but carboxylic acids are more than ten billion (1010) times stronger acids than alcohols. Why should a carbonyl (C=O) group adjacent to a hydroxyl group have such a profound effect on the acidity of the hydroxyl proton?
When an acid donates a proton to another species (for example, to an OH− ion), a carboxylate anion, RCOO−, is formed. The more stable the carboxylate ion is, the more readily the proton can be donated. Consequently, anything that stabilizes the anion (A–) of an acid makes that acid (H–A) stronger. A carbonyl group allows the carboxylate anion to be stabilized by resonance, as shown in Figure 1. The two contributing structures have equal weight in the resonance hybrid, and extra electron (the overall negative charge in the anion) is delocalized to both oxygen atoms. This leads to a markedly increased acidity. In contrast, there is no such resonance stabilization for the anion of an alcohol, the alkoxide anion, where the excess electron is localized to the single oxygen atom.
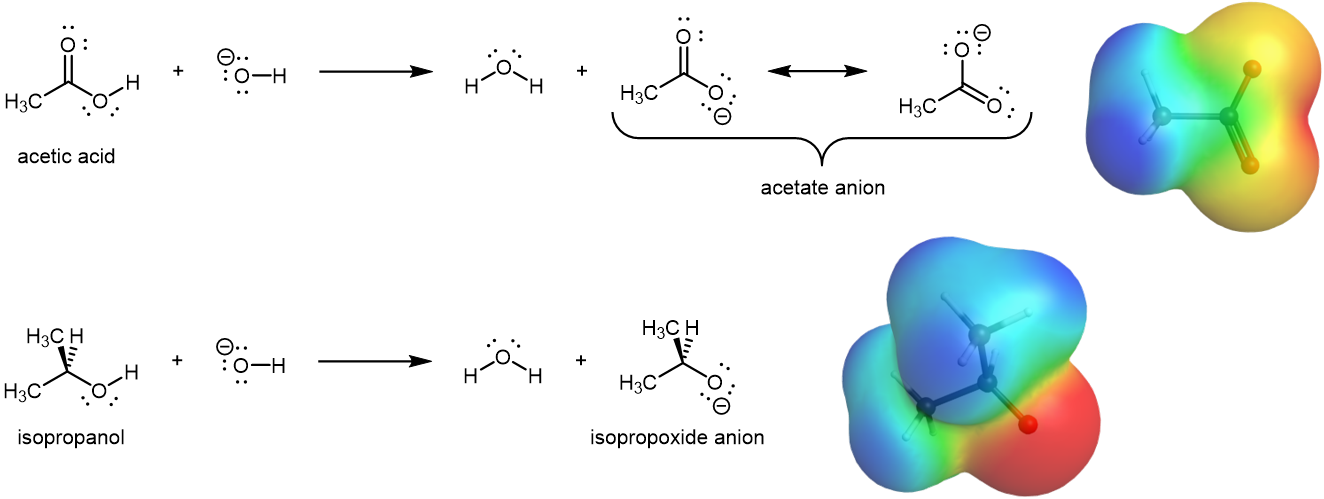
D15.2 Esters
The odor of ripe bananas and many other fruits is due to the presence of esters, compounds that contain a carbonyl group (C=O) and a second oxygen atom bonded to the carbonyl carbon and to another carbon atom (see Figure 2).
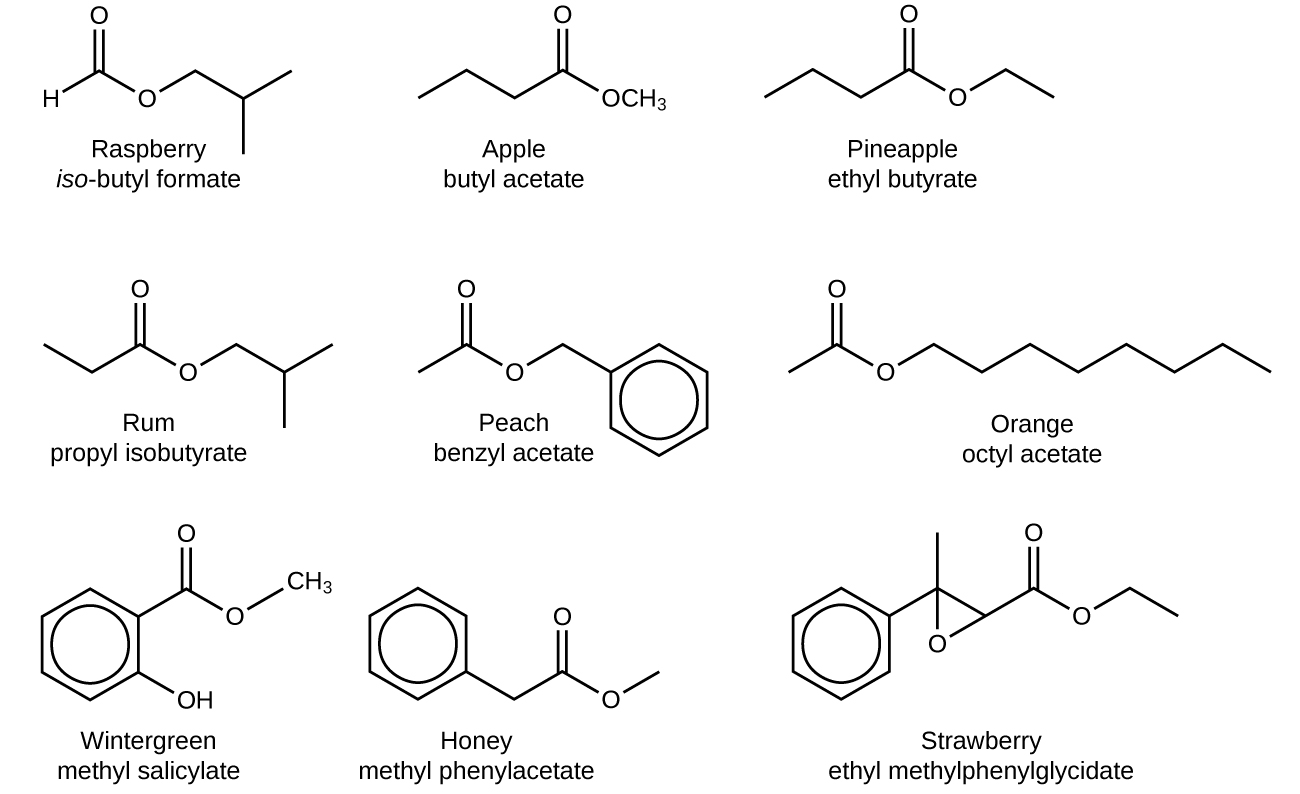
An ester can be produced from a carboxylic acid and an alcohol by a condensation reaction. In a condensation reaction, two smaller molecules join together to form a larger molecule while giving off a very small, stable molecule such as H2O. For example, ethyl acetate, CH3COOCH2CH3, is formed when acetic acid reacts with ethanol:
Condensation reactions are reversible; that is, not all of the reactant substances is converted to product substances; if products are mixed, some reactants will form. The reverse of condensation is called a hydrolysis reaction. In hydrolysis, water breaks apart the larger molecule: hydro, “water”; lysis, “breaking apart”.
Although the ester functional group has a polar carbonyl, it contains no hydrogen atoms suitable for hydrogen bonding. Therefore esters have lower boiling points relative to alcohols and carboxylic acids of similar molecular size. Ethyl acetate, for example, boils at 77.1°C, lower than 1-pentanol at 138 °C, as well as ethanol (78.5°C) and acetic acid (117.9°C).
D15.3 Amines
Amines are similar to ammonia except amines contain carbon-nitrogen bonds.
You can group amines by the number of carbon atoms bonded to the nitrogen. In the above example, methylamine is a primary (1º) amine, dimethylamine is a secondary (2º) amine, and trimethylamine is a tertiary (3º) amine. (Note the difference from 1º, 2º, and 3º alcohols, where the number of other C atoms bonded to the carbon with the –OH group that determines primary, secondary, and tertiary.)
The trigonal pyramidal geometry of amines makes them polar molecules. 1º and 2º amines are capable of strong hydrogen bonding due to the presence of a N-H bond and the lone pair on the N atom, but 3º amines cannot form hydrogen bonds. For example, for the three amine isomers shown below, the boiling point of the 3º amine is significantly lower than the 1º and 2º isomers.
Like ammonia, amines are basic due to the lone pair of electrons on their nitrogen atoms, and can undergo acid-base reactions to form protonated amine cations:
D15.4 Amides
Amides have molecules that contain nitrogen atoms connected to the carbon atom of a carbonyl group. Like amines, amides can be classified by the number of carbon atoms bonded to the nitrogen.
There is a crucial difference between amines and amides—there is an important resonance structure for an amide molecule that shows that the lone pair on nitrogen is involved in the π bonding network (Figure 2). Therefore, the amide N atom is sp2 hybridized and has a trigonal planar geometry.
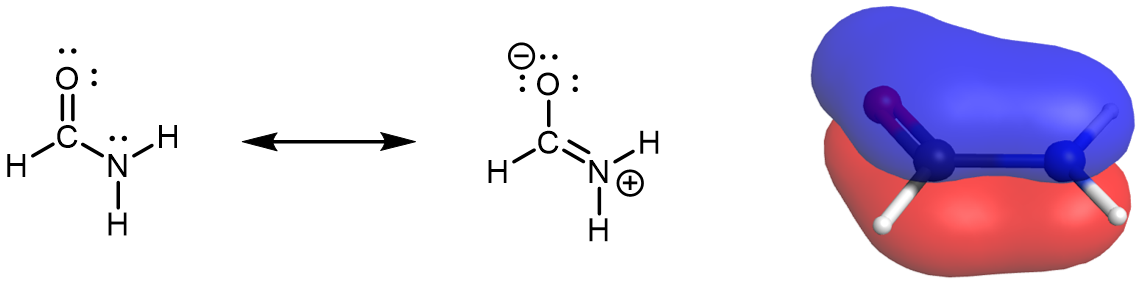
Moreover, because the lone pair on N atom is part of the π bonding network, it is not available for hydrogen bonding nor is the N atom basic. Hydrogen bonds can still form in 1º and 2º amides between the hydrogen from the N-H bond and the lone pairs on the O atom,. Because the lone pair in a p orbital and part of the π bonding network amides are about 1010 times less basic than amines.
Amides can be produced via condensation reactions starting from carboxylic acids and 1º or 2º amines (or ammonia):
It is through this reaction that amino acids (molecules containing both an amine and a carboxylic acid functional group) link together in a polymer to form peptides and proteins.
Exercise 1: Recognizing Functional Groups
Exercise 2: Structures of Amines
Exercise 3: Names and Functional Groups
Enter the name for each molecule shown below. (You can copy and paste from the list.)
List of names:
- ethanamide
- ethylamine
- ethylaminol
- 1-methylbutanone
- methyl propanal
- methyl propanoate
- methyl propanoic acid
- propanoic acid
- propyl methanoate
- 2,2,2-trichloroethanal
- 2,2,2-trichloroethanoic acid
- 2,2,2-trichloropropanoic acid
D15.5 Addition Polymers
Polymers (also called macromolecules) are large molecules made by combining many covalently-linked repeating units called monomers. Polymers can be natural (such as starch and proteins) or synthetic (such as nylon and polyethylene). Because they include a variety of structures and functional groups, polymers have a broad range of properties and uses that make polymer-based plastics integral parts of our everyday lives.
Addition polymers are made by addition reactions involving many monomer molecules. In an addition reaction, two molecules combine to form a single product molecule (see Section 8.3, which describes addition to a double bond). Typical monomers for addition polymerization have at least one double bond. Radical addition polymerization is initiated by a molecule that is a free radical initiator. This is often an organic peroxide, R–O–O–R, which can form two free radicals by breakage of the –O–O– bond. When each radical encounters a monomer, the two electrons in the monomer π bond unpair and a new free radical forms. That new radical adds to another monomer, lengthening the chain, and the process repeats with many, many more monomers—sometimes as many as 100,000 units.
Figure 3 shows the example of ethylene (H2C=CH2) monomers polymerizing in the production of polyethylene.
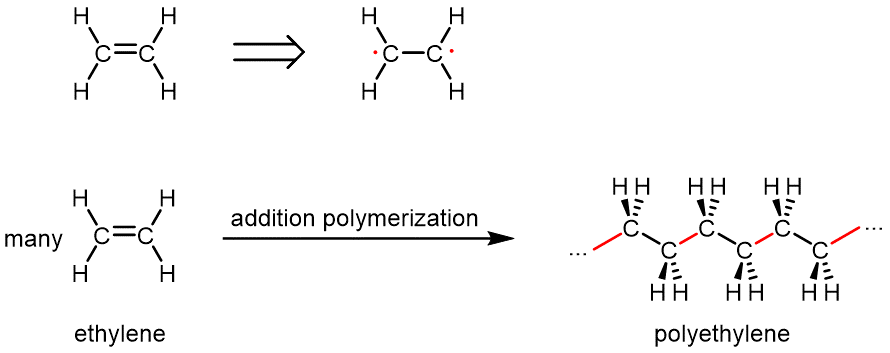
In polyethylene, all of the ethylene double bonds have been turned into single bonds. No atoms have been lost and you can see that the monomers have just been joined in the process of addition. The polymer at a molecular level consists of a collection of long-chain alkane molecules, most of which contain tens of thousands of carbon atoms. Depending on the type of catalyst used, the polymer molecules can be very long linear chains or there can be branches in the chains. This allows fine tuning of the properties of plastic materials made from the polymers.
Many of polyethylene’s properties are what we would expect from its molecular composition. The fact that it is a mixture of molecules each of slightly different chain length explains why it softens over a range of temperatures rather than having a single melting point. Because the long polymer strands are held together by only London dispersion forces, melting and softening occurs at relatively low temperatures (considering their extensive lengths) and polyethylene is soft and easy to scratch.
London dispersion forces also explain why different types of polyethylene have different properties. LDPE (Low Density PolyEthylene), produced using peroxide catalysts at 500 °C and 1000 atm, is a transparent polymer with highly branched chains which do not pack together well. The looser packing (and hence lower density) leads to weaker London dispersion forces between polymer strands, and LDPE makes for a very flexible plastic. Today most LDPE is used for plastic sheets for packaging and trash bags and flexible snap-on lids.
HDPE (High Density PolyEthylene), produced using Ziegler-Natta aluminum titanium catalysts, has very little branching, allowing the polymer strands to pack closely and have stronger London dispersion forces. HDPE is three times stronger than LDPE and more opaque. About 45% of the HDPE is molded into milk and disposable consumer bottles. HDPE is also used for crinkly plastic bags to pack groceries at grocery stores.
Podia Question
The solubility in water at 20 °C is given for substances consisting of each of the molecules shown below. List the functional groups that are present in each molecule. Relate the solubility data to molecular structure and functional groups. Explain each substance’s relative solubility using scientifically accurate language.
Two days before the next whole-class session, this Podia question will become live on Podia, where you can submit your answer.